This brief discusses policies needed to ensure equitable and universal access by all those in need globally to future vaccines for SARS‑Cov‑2, the virus that causes COVID‑19, and treatments for the disease. It provides a snapshot of the vaccine and drug candidates in the current R&D pipeline. It then discusses the need for international co-operation to focus on three critical issues which are beyond the initial phases of clinical research. First, there is a need for pull mechanisms to incentivise the swift completion of the most promising R&D projects, and avoid that they are abandoned mid-way should the pandemic subside. Second, large-scale manufacturing capacity has to be built even before we know which candidates will be successful. This is particularly important for vaccines to ensure timely production of a large number of doses needed, and could also be achieved through designing appropriate pull mechanisms. Third, rules need to be set now to manage intellectual property rights and procurement to ensure equitable access, affordability and supply in sufficient quantities.
Treatments and a vaccine for COVID-19: The need for coordinating policies on R&D, manufacturing and access
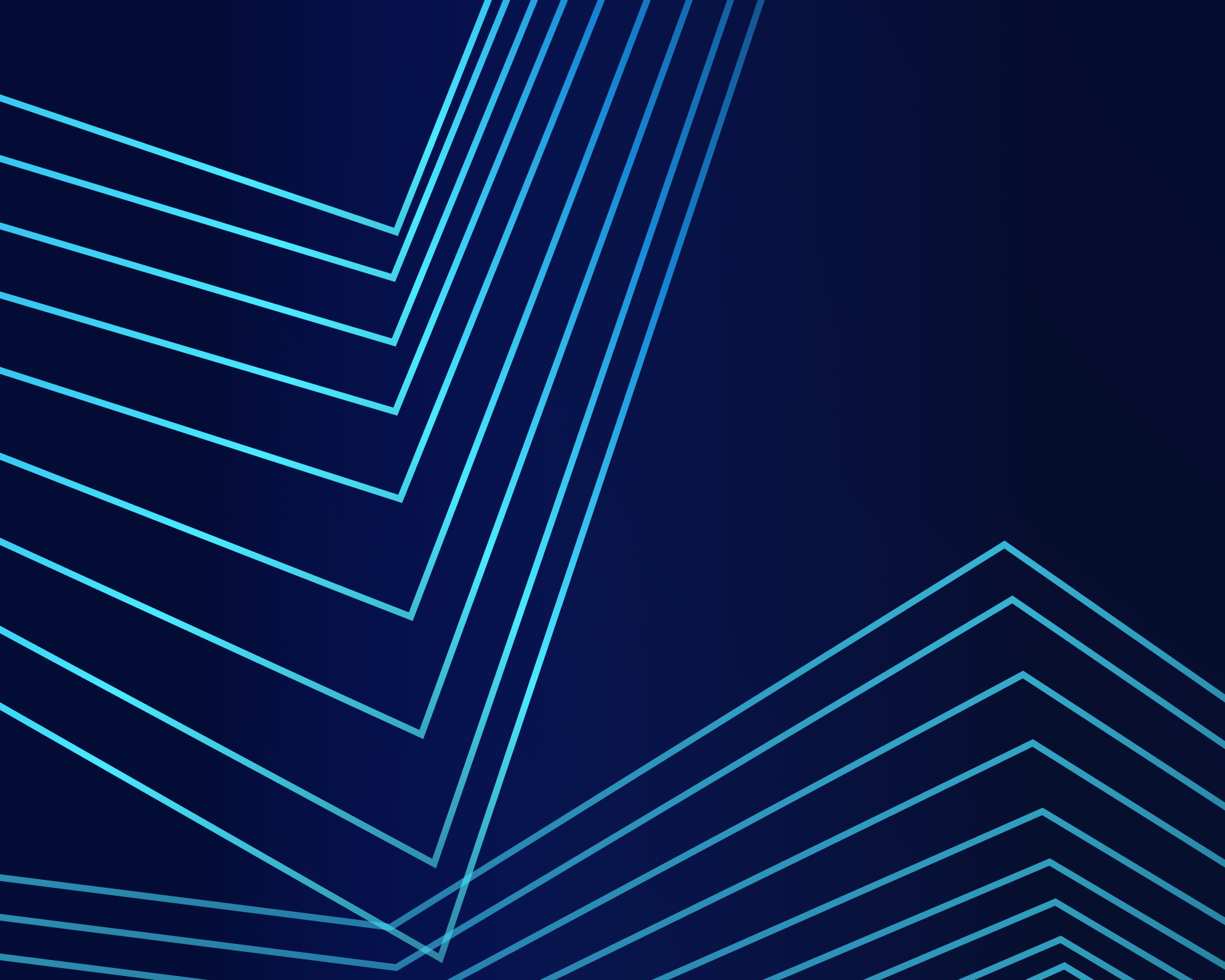
Abstract
Key findings
Based on the most optimistic estimates, it will take at least 12 to 18 months for an effective vaccine for SARS‑CoV‑2 to become widely available. However, this assumes that one of the candidates currently in clinical trials turns out to be successful. Based on experience with the development of other vaccines, it could well take several years for a vaccine to be available.
Attention has so far focussed almost exclusively on promoting research and early stage development of vaccines. But scientific breakthroughs – necessary though they are – are not enough. To end the pandemic, health systems have to vaccinate 50 to 75% of the global population. This requires building manufacturing and distribution capacity, making a new vaccine affordable, deciding who should get access first and planning massive vaccination campaigns. Policy has only recently been refocused on building capacity in some countries, but not in a co-ordinated way, which is a mistake.
Pull mechanisms, such as an international Advance Market Commitment (AMC), are needed to complement the funds already allocated to provide incentives for completing R&D and making a vaccine available. While push mechanisms, such as research grants, pay directly for R&D to ensure that it is conducted, they are not conditional on a specific outcome. Pull mechanism, on the other hand, reward successful completion of R&D and reduce uncertainty around returns to investment. They harness competition, incentivising private investors to join a race for developing and producing effective health technologies. An international AMC would also help attract private capital for building manufacturing and logistical capacity.
International co-operation between governments is urgently needed to project demand and plan the necessary capacity to produce sufficient quantities of a future vaccine that will turn out to be effective.
Medicines to treat COVID‑19 have so far provided disappointing results and no cure is yet in sight. Progress is mainly being made by repurposing existing medicines but most of them are associated with significant side effects and target narrow patient groups, such as the most severe cases. The large-scale trials that can provide robust evidence are yet to deliver results. Eventually, the treatments currently tested may contribute to a “tool box”, from which doctors would choose to manage patients on a case-by-case basis.
Although there are differences to vaccines, many of the same challenges in building capacity and ensuring population access also apply to medicines for treating COVID‑19. International pharmaceutical supply chains need to be reinforced to facilitate the arrival of these products.
Governments also need to ensure that a future vaccine and effective treatments will be widely accessible. They need to agree upfront on rules for intellectual property rights (IPRs) and procurement to avoid bidding wars between countries and high prices, which could prevent the most vulnerable from having access. Public funding, whether allocated through push or pull mechanisms, should be tied to conditions for accessibility and affordability. Governments also need to agree on how to allocate scarce product volumes between them based on need.
The outbreak of SARS-CoV‑2, the virus that causes COVID‑19, poses an unprecedented challenge to economies and societies. Safe and effective vaccines that provide immunity to populations, and effective treatments for those who are infected and develop symptoms of COVID‑19, are the only long-term solutions that will allow societies and economies to return to normal life without unacceptable loss of life. While the R&D effort so far has been impressive, many hurdles still have to be cleared before a vaccine and treatments are widely available. International co-operation is key to accelerate progress and must focus on preparing manufacturing and logistical capacity, as well as the conditions for these innovations to be affordable and accessible to populations around the world.
Since the global outbreak in early 2020, a wide range of researchers, backed by public and private funders, have rushed to accelerate R&D on vaccines and treatments. As a result, pre-clinical research and clinical trials have increased significantly. Hundreds of clinical trials have been registered since the beginning of 2020. Most of them test drug candidates but several vaccine candidates are also being tested. Testing many different approaches can increase the chances that at least one, or a few, candidates are successful. However, too much un-coordinated experimentation and a lack of adherence to shared standards in research is not only a waste of resources and time by tying up researchers and patients in clinical trials that are not conclusive, but can also impede the generation of robust evidence to underpin medical knowledge.
Clinical trials of vaccine candidates were well underway but an effective vaccine might not be available soon
Ten vaccine candidates were in clinical trials by May 2020 and many more were in pre‑clinical stages
As of 26 May, at least ten vaccine candidates were in clinical trials (see Table 1). Five candidates were in phase 1 trials and five candidate in combined phase 1 and 2 trials. The first phase 1 trial1 is expected to be completed before the end of 2020. In addition, as many as 160 candidates were estimated to be in exploratory and pre-clinical R&D phases (LSHTM Vaccine Centre, 2020[1]), which precede clinical trials. The number of candidates under investigation is increasing rapidly.
Table 1. Overview of SARS‑CoV‑2 vaccine candidates currently in clinical trials
Status per 26 May 2020
Sponsor (Country) |
Candidate name |
Vaccine platform (see Table 2) |
Current trial phase |
Expected completion date of current trial(s) |
---|---|---|---|---|
Sinovac (China) |
Unknown |
Inactivated |
Combined 1 and 2 |
Mid 2020 |
Inovio Pharmaceuticals (United States) |
INO‑4800 |
DNA |
1 |
Late 2020 |
CanSino Biological Inc. and Beijing Institute of Biotechnology (China) |
Ad5‑nCOv |
Viral vector |
Combined 1 and 2 |
Early 2021 |
BioNTech, Fosun Pharma and Pfizer (China, Germany, United States) |
BNT‑162 |
mRNA |
Combined 1 and 2 |
Mid 2021 |
Moderna and United States NIAID (United States) |
mRNA‑1273 |
mRNA |
1 |
Mid 2021 |
University of Oxford Jenner Institute (United Kingdom) |
ChAdOx1 |
Viral vector |
Combined 1 and 2 |
Mid 2021 |
Beijing and Wuhan Institutes of Biological Products and Sinopharm |
Unknown |
Inactivated |
Combined 1 and 2 |
Late 2021 |
Symvivo (Canada) |
bacTRL-Spike |
DNA |
1 |
Late 2021 |
Shenzhen Geno-Immune Medical Institute (China) |
LV-SMENP-DC |
Other |
1 |
2023 |
Shenzhen Geno-Immune Medical Institute (China) |
Pathogen-specific aAPC |
Other |
1 |
2023 |
Note: The status of the development pipeline is evolving rapidly. Information in this table may be out-of-date quickly.
Source: Authors based on EU Clinical Trials Register (EMA, 2020[2]), LSHTM Vaccine Centre (2020[3]), United States NIH Clinical Trials Register (2020[4]).
Vaccines can be of different types or “platforms”, described in Table 2. The various platforms have both advantages and disadvantages, and affect the expected duration of R&D and manufacturing processes. Nucleic acid vaccines deliver genetic instructions (in the form of DNA or RNA) coding for a viral protein that prompts an immune response. The nucleic acid is inserted into the host’s cells, which then produces copies of the virus protein. These vaccines are safe and easy to develop and their production only involves producing genetic material. Yet, they are still unproven since no human vaccine approved for marketing so far relies on this platform. Recombinant protein vaccines are made of viral proteins, produced by genetic engineering, which are intended to stimulate the host’s immunity. In order to work, these vaccines may require multiple doses and the use of adjuvants, which are immune stimulating molecules delivered alongside the vaccine. Viral-vector vaccines use a genetically engineered virus (such as an adenovirus) to produce proteins of the targeted virus in the body of the host. These viruses are weakened so they cannot cause disease. Viral-vector vaccines tend to be safe and provoke a strong immune response but existing immunity against the viral vector can reduce their efficacy. Finally, live attenuated and inactivated virus vaccines use the virus itself, in a weakened (attenuated) or inactivated form, to generate immunity. Many existing vaccines are made in this way today, such as those against measles and influenza, but they require extensive safety testing.
The SARS‑CoV‑2 vaccine platforms currently in phase 1 and 2 clinical trials are nucleic acid vaccines, including DNA and messenger RNA (mRNA, an intermediary between DNA and proteins), viral vector-based vaccines and inactivated vaccines. Candidates currently in pre-clinical R&D also include recombinant protein and live-attenuated vaccines and modifications of vaccines that have already been tested against other diseases, such as measles.
Table 2. Vaccine platforms considered for the development of a vaccine against SARS‑CoV‑2
Platform |
Description |
Approved human vaccines using this platform? |
Pros in SARS‑CoV‑2 context |
Cons in SARS‑CoV‑2 context |
---|---|---|---|---|
Nucleic acid vaccine (RNA vaccine) |
Inject RNA encoding the antigen or antigens against which an immune response is sought. The body’s own cells use this genetic material to produce the antigens. |
No |
Potentially broad and long-term immune responses, excellent stability and relative ease of large-scale manufacture. No infectious virus needs to be handled, vaccines are typically immunogenic, rapid production possible. |
Still unproven in humans. Safety issues with possible reactogenicity. |
Nucleic acid vaccine (DNA vaccine) |
Inject DNA encoding the antigen or antigens against which an immune response is sought. The body’s own cells use this genetic material to produce the antigens. |
No |
Potentially broad and long-term immune responses, excellent stability and relative ease of large-scale manufacture. No infectious virus needs to be handled, easy scale up, low production costs, high heat stability, tested in early stage clinical trial for SARS-CoV‑1, rapid production possible. |
Still unproven in humans. Vaccine needs specific delivery devices to reach good immunogenicity. |
Recombinant protein vaccines |
Inject only components of the pathogen, or antigens, that best stimulate the immune system, produced in‑vitro by cells into which the genetic code for the viral protein has been inserted. |
Yes, for influenza, human papillomavirus (HPV) and hepatitis B (HBV) |
Can be safe and easy to produce, using various techniques. No infectious virus needs to be handled, adjuvants1 can be used to increase immunogenicity. |
Often requires the incorporation of adjuvants1 to elicit a strong protective immune response. Global production capacity might be limited. Some types of these vaccines are difficult to develop (e.g. virus-like particles). |
Viral vector-based vaccines |
Inject only components of the pathogen, or antigens, that best stimulate the immune system. Use a harmless virus or bacterium as a vector, or carrier, to introduce genetic material into cells. |
Yes, for vesicular stomatitis virus (VSV) and Ebola |
Can be safe and easy to produce, using various techniques. No infectious virus needs to be handled, excellent preclinical and clinical data for many emerging viruses, including MERS-CoV. |
Often requires the incorporation of adjuvants1 to elicit a strong protective immune response. Vector immunity might negatively affect vaccine effectiveness (depending on the vector chosen). |
Live attenuated vaccines |
Inject a weakened form of the germ that causes a disease, similar to the natural infection they help prevent. |
Yes, for measles, mumps, and rubella (MMR) and rotavirus |
Create a strong and long-lasting immune response. Straightforward process used for several licensed human vaccines, existing infrastructure can be used. |
Creating infectious clones for attenuated coronavirus vaccine seeds takes time because of large genome size. Safety testing needs to be extensive. |
Inactivated vaccines |
Inject an inactive or dead version of the germ that causes the disease. |
Yes, for influenza |
Straightforward process used for several licensed human vaccines, existing infrastructure can be used, has been tested in humans for SARS-CoV‑1, adjuvants1 can be used to increase immunogenicity. |
Usually do not provide immunity as strong as that induced by live vaccines, requiring several doses over time (booster shots). Large amounts of infectious virus need to be handled (could be mitigated by using an attenuated seed virus). |
1. Adjuvants are immune stimulating molecules delivered alongside the vaccine.
Source: Adapted from Amanat Fatima (2020[5]), “SARS-CoV‑2 vaccines: status report”, https://doi.org/10.1016/j.immuni.2020.03.007.
However, a vaccine may not be widely available for months or years from now
Based on information currently available, reasonable estimates of the time it will take for a vaccine for SARS‑CoV‑2 to become available range anywhere from 12/18 months to several years. The assumption that a vaccine could be widely available within months is optimistic and would be much faster than the historical average time of 6 to 9 years for the completion of the clinical development of a novel vaccine (see Box 1).
Development of vaccine candidates for SARS‑CoV‑2 has started at breakneck speed in 2020. Estimates on the optimistic end of the range assume that one of the candidates already in clinical trials will turn out to be safe and effective. For a number of reasons, development might indeed be quicker than usual.
First, the genetic information of viruses can be sequenced much faster than before and SARS‑CoV‑2 is genetically similar to coronaviruses subject to prior research, so vaccine research and development does not start from scratch.
Second, a large number of candidates are being tested in parallel, using different platforms, which increases the chances that at least one might be successful.
Third, some types of candidates in development rely on a new platform: messenger nucleic acid vaccines (mRNA), which have great potential for rapid development (see, Tables 1 and 2 and Thanh Le et al. (2020[1])). Others may build on existing vaccines or candidates in development for other diseases, so some relevant evidence of safety data may already be available.
Fourth, the current pandemic makes development urgent. This could be leading to faster development processes, for instance through shorter clinical trials, running trials in parallel that are usually done sequentially, and faster regulatory approval.
However, there are also limits to accelerating the clinical development process and acceleration cannot come at the expense of safety. Safety standards have to be high for vaccines and treatments, and particularly for vaccines because they are injected into a large number of healthy people (Jiang, 2020[6]). There thus has to be robust evidence that their benefits outweigh the risks. Knowledge about immunity is still limited. Inducing a desired immune response against SARS‑CoV‑2 might be particularly challenging because of the risk of harmful inflammatory and autoimmune reactions (Iwasaki and Yang, 2020[7]). Given the uncertainties, it is very difficult to estimate with more precision how long it will take for an effective and safe vaccine to become available.
It will take at least 12 to 18 months for an effective #vaccine against #COVID19 to become widely available, and only if current clinical trials turn out to be successful
Box 1. Duration and success probabilities of clinical trials
Despite encouraging early progress on the development of a vaccine for SARS-Cov‑2, past experience serves as a guide to temper expectations while maintaining, if not further reinforcing, the efforts. Vaccine development involves complex genetic and biological engineering. Testing a vaccine requires a sufficient number of people that are exposed to the disease and/or a valid surrogate measure of immunity. Following a pre-clinical phase that can last several years and involves identification, characterisation and synthesis of the antigen, i.e. the substance which induces an immune response in the body, and/or its genetic information, and lab and animal testing, clinical trials typically also take years to complete. Estimates of the probability of approval of vaccines that enter clinical trials range from 12 to 33%, after some 7 to 9 years of testing. Medicines can be relatively straightforward to produce but also require lengthy trials. A typical successful drug candidate for infectious diseases undergoes approximately 7 years of trials, of which 2 to 3 years are needed for phase 3 trials of efficacy alone, and only 1 in 4 candidates that enters trials is approved.
Source: Authors based on Pronker et. al. (2013[8]), “Risk in Vaccine Research and Development Quantified”, https://doi.org/10.1371/journal.pone.0057755; Wong, Siah and Lo (2018[9]), “Estimation of clinical trial success rates and related parameters”, https://doi.org/10.1093/biostatistics/kxx069.
Despite impressive initial R&D efforts, international co-ordination is even more critical to complete R&D and ensure universal access
Many hurdles still have to be cleared before a vaccine can be widely delivered to populations. Once a vaccine is authorised for marketing, this does not mean that it is accessible to populations. As shown in Figure 1, capacity has to be built so that sufficient doses can be manufactured and doses have to be priced affordably, procured by various public and private purchasers across countries, and finally delivered to the population through health systems. This is a formidable task. Assuming a basic reproduction number, also referred to as R0, of 2 to 4,2 models of the spread of COVID‑19 in European countries estimate that at least 50% to 75% of people need to be immune for a population to reach herd immunity (Flaxman et al., 2020[22]). This implies that, globally, 4 to 6 billion people will need to be vaccinated.
If there is not to be a delay between getting regulatory approval, and getting vaccines to those who need them, then for COVID‑19 many of the activities that usually occur after R&D and marketing authorisation will have to be planned now and executed in parallel to R&D activities.
Getting the processes, which usually occur after marketing authorisation (see the red box of in Figure 1), ready now requires strong international co-ordination. Vaccine development is currently still in the early stages of this entire process, as shown by the double blue line in Figure 1. At this stage, it is still very risky for firms to build manufacturing capacity. But if capacity is not built before marketing authorisation, it might take several months or years after authorisation for sufficient capacity to be available, with enormous costs to society in terms of forgone health and economic activity. Likewise, health systems cannot afford to only start thinking about procurement and delivery of a vaccine to populations once it can be purchased. This needs to happen now.
Figure 1. It is a long way from R&D to population access

New pull mechanisms should complement existing push mechanisms and more co‑ordination is needed to complete promising R&D projects
A combination of push and pull mechanisms are needed to complete development of a vaccine, not only to respond to the current pandemic but also to prepare for potential future disease outbreaks. They should be designed to help ensure that the R&D projects that are currently in early clinical phases are brought all the way to successful conclusion and not abandoned once the immediate urgency of the pandemic has subsided. Issues around the sustainability of R&D funding have been reported for prior disease outbreaks. For instance, the vaccine R&D projects launched after the outbreak of SARS-CoV‑1 in 2003 were abandoned before successful completion due to a lack of resources. This is despite the availability of a number of promising candidates and awareness of the risk these pose on humans (Chen et al., 2020[20]; Menachery et al., 2015[21]).3 Had these been completed at the time, it would have given current R&D on SARS‑CoV‑2 vaccines a head start. Completion of current vaccine development might also have relevance beyond response to the current pandemic if COVID‑19 turns into a seasonal illness, similar to influenza.
Push mechanisms, such as research grants, ensure that R&D is conducted but are not conditional on a specific outcome. While they are an effective means of kick-starting early stage R&D, they do not provide incentives for completing R&D through expensive late-stage phases and bringing new products to market. Pull mechanisms can overcome some of these problems by rewarding successful completion of clinical trials, getting a product approved for marketing by regulatory agencies, or delivering a specified quantity of an approved product. They can harness competition and can be more efficient than push mechanisms, by incentivising private investors to “enter a race” for developing and producing effective health technologies. Examples of pull mechanisms are when governments or philanthropists commit to awarding a prize to the first firm or research institution to develop a new product, or guarantee the purchase of a specified volume of a new vaccines once it has been shown to be effective and is approved by regulatory agencies. Public and private not-for-profit funding play a significant role in catalysing progress in R&D towards vaccines (see Box 2) and towards new medicines for infectious diseases (Kremer, 2004[13]). But pull mechanisms require significant financial commitments to be effective. Push and pull mechanisms are discussed below.
Box 2. Financing vaccine R&D
In general, the scientific and technical complexities in production and the need for extensive clinical trials make the development of vaccines not only risky but also expensive. Estimates of the average cost of developing a new vaccine vary widely, but are in the magnitude of several hundred million USD to USD 1 to 2 billion (Gouglas et al., 2018[14]).
Both private and public funding can play a role in R&D but some vaccine markets are not very attractive for private for‑profit investment. The latter typically occurs when there is a prospect of a sufficiently large return and there are good chances that this return will actually materialise. However, unless successive boosts are necessary, the demand for vaccine doses disappears once a population has gained immunity or the disease is no longer perceived as a threat. This dynamic makes returns on investment uncertain. As with other medical technology, the possibility that R&D fails is another source of uncertainty. In contrast to medicines, however, vaccines are not only expensive to develop but can also be expensive to produce. At the same time, their potential to protect entire societies from infectious disease outbreaks is only realised if a large share of the population is immunised. So vaccines also have to be priced modestly to allow for broad population access, which means that profit margins may be low and insufficient to compensate for uncertainty. Taken together, these factors reduce the prospect of attractive returns from vaccine R&D for private investors.
The Coalition for Epidemic Preparedness Innovations (CEPI), a public‑private partnership that funds vaccine R&D and to which governments of several OECD countries already contribute, estimates that USD 2 billion will be required to complete R&D of an effective vaccine for SARS‑CoV‑2. CEPI has raised just over USD 1 billion by 26 May.4 A plethora of other entities have pledged funding, including various governments of OECD countries, non-governmental organisations and the pharmaceutical industry. On 24 April, the WHO launched the Access to COVID‑19 Tools (ACT) Accelerator, a global collaboration between public and private entities to accelerate not only R&D but also to ensure equitable global access to safe and effective COVID‑19 diagnostics, treatments and vaccines.5 The Coronavirus Global Response led by the European Commission has so far raised EUR 9.8 billion in funding, including pledges by many OECD countries, to support WHO ACT, CEPI and other efforts.6
With the vast amounts of funding that have been pledged, efficient ways of allocating resources have to be found by striking the right balance between an approach of “letting a thousand flowers bloom” in R&D, on the one hand, and more co-ordination and co-operation, on the other. There are various mechanisms to allocate public or non-profit funding, some of which help “de-risk” investments to attract private for-profit capital.
At an early stage, many disparate projects increase the chances of success. This is because most vaccine and drug candidates are expected to fail so the chances of success of any single candidate is low. In-vitro research, early-stage laboratory studies and small clinical trials are also relatively inexpensive compared to larger trials later on. As R&D progresses and clinical trials become larger and more expensive, it makes more sense to co-operate, pool resources and allocate them to the most promising projects. As discussed below, the amount of money necessary for an effective pull mechanism for a SARS‑CoV‑2 vaccine could well be beyond the funding capacity of a single national government. International co-operation could make the amounts committed large enough for such a mechanism to be effective.
COVID‑19 R&D funding has so far been allocated through push mechanisms, an effective means of stimulating R&D in early stages
Much of the public R&D funding for COVID‑19 has so far been allocated through direct research grants, one type of so‑called push mechanisms, which ensure that R&D is conducted but which are not conditional on a specific outcome. Push mechanisms are an effective and straight-forward means of boosting particular lines of research. At an early stage of R&D, having funders back many disparate research projects through push mechanisms, including grants to research institutions and subsidies to biotech and pharmaceutical firms, can be an effective way of making progress. Another example of a push mechanism, typically related to later R&D stages, are product development public-private partnerships (PD-PPPs). They harness the strengths of both public and private sectors and make strategic and targeted investments for certain research centres to develop a pipeline of drugs or vaccines. The public sector often contributes with funding and academic research, while the private sector brings expertise in business, clinical trials, supply chains and intellectual property (Widdus, 2001[12]; Widdus et al., 2001[13]). Philanthropic organisations usually provide financial support for such arrangements. Examples of these arrangements are the Global Alliance for Vaccine Initiative (GAVI), Malaria Vaccine Initiative (MVI), and the International AIDS Vaccine Initiative (IAVI).7
In addition, push mechanisms can provide predictable and long-term funding for fundamental science and its applications to R&D. For instance, the underlying basic research for the first Ebola vaccine, which was authorised in 2019, was conducted long before the 2014 outbreak and catalysed investment in clinical trials. Investment in basic research accelerates product development and helps prioritise preparedness actions for potential future pandemics. Examples of such initiatives are the WHO Disease X, part of the R&D Blueprint, to anticipate future outbreak caused by unknown pathogens (Simpson et al., 2020[14]), the Global Virome Project aiming at discovering 500 000 new viral threats, and the ONE Health European Joint Programme, which explores the relationship between humans, animals and environment.8
While push mechanisms can stimulate R&D in areas where there is a gap between private and social returns to R&D, they present a number of issues. First, they require funders to decide ex-ante which scientific approaches are most promising and worth pursuing without having full information about the prospects of the alternative approaches (Widdus et al., 2001[13]). There is thus an information asymmetry between funders and research institutions. This may lead to over-investment in R&D projects that are unlikely to be successful. Second, if funders fully fund the cost of research inputs rather than outputs, they bear the entire risk of failure. Third, because researchers do not bear the risk of failure, push mechanisms do not incentivise speed and efficiency of research. Researchers have incentives to exaggerate the prospects that their approach will succeed and to advance their careers to secure future funding (Morel and Mossialos, 2010[18]; Mossialos et al., 2010[19]).
Pull mechanisms should also be used
Funders should complement push with pull mechanisms that make the disbursement of funding conditional on the achievement of relevant R&D results. Pull mechanisms essentially operate through reducing the uncertainty around returns to investment for private investors and incentivise investors to join a race for developing and producing an effective health technology. Advance market commitments (AMC) and innovation prizes are two examples of pull mechanisms that can help mobilise private investment in COVID‑19 R&D and incentivise researchers to self-select the most promising projects:
In an Advance Market Commitment (AMC), buyers commit in advance to purchasing a specified volume of a health technology still in development if it meets specified criteria and at a guaranteed price. AMCs therefore not only incentivise R&D but also the production and delivery of the final product, because funds are only disbursed upon its purchase. Once the guaranteed volume is purchased, the manufacturer is contractually obliged to supply further volume at a lower price. There is thus a two‑stage pricing system: one relatively high price that is guaranteed up to a fixed volume purchased, which provides a risk-adjusted return to the R&D investment made by the producer, and a second, lower price set at a level closer to the cost of production (the “base price”). Specified criteria in the commitment include technical requirements like the disease to be prevented or treated, the target population, minimum efficacy, dosage, route of administration, storage, quality and safety requirements. AMCs can also specify conditions for procurement, licensing of intellectual property rights (IPRs) and affordability or access. Prior examples of AMCs have been proposed to incentivise the development of a pneumococcal vaccine (Levine, Kremer and Albright, 2005[19]) and a treatment tackling tuberculosis related drug-resistance (Chalkidou et al., 2020[20]).
Innovation prizes commit funders to disburse a pre-specified sum upon achievement of a specified milestone related to the development of a health technology. Milestones can be defined to incentivise product quality and effectiveness. They can be intermediate, such as reaching a pre-defined clinical trial result, or more final, such as marketing authorisation of an effective vaccine.
An effective AMC for a SARS‑CoV‑2 vaccine would require strong international co-operation among governments to commit funding in the order of several tens of billions, in combination with push mechanisms to support the building manufacturing capacity. To ensure that a vaccine is developed and can be manufactured at scale within months, the amount committed would need to provide the prospect of a risk-adjusted return large enough to induce several firms to invest in parallel, including firms with vaccine candidates that have individually a small probability of success. However, because such an AMC would incentivise private investment and would only disburse funding to effective vaccines at a pre-specified price, it could eventually be cheaper for governments than funding many different R&D projects through push mechanisms, including those that fail. This may be especially true if governments subsequently find themselves engaging in a bidding war to purchase sufficient doses of the successful vaccine. Crucially, an AMC incentivises speed in development and production of a vaccine – and the economic returns for societies of having a vaccine earlier are much higher than the cost of an AMC. The Global Alliance for Vaccine Initiative (GAVI) has suggested that funds raised for a vaccine by the WHO ACT Accelerator could be allocated through an AMC.9 The GAVI pilot AMC for the pneumococcal vaccine, which has allowed for immunisation of 149 million children by 2018 (GAVI AMC Secretariat, 2019[22]), provides an example of how an AMC can function in practice. How an AMC for a SARS‑CoV‑2 vaccine might work is described in Box 3.
Governments must work together to ensure that a #vaccine for #COVID19 will be widely accessible. Agreement on intellectual property rights and procurement is needed so that bidding wars between countries do not stop people getting the vaccine
Box 3. How might an advance market commitment (AMC) for a SARS‑CoV‑2 vaccine work?
A group of economists from the United States designed a model that estimates the amount of funding required for an effective international advance market commitment (AMC) for a vaccine against SARS‑CoV‑2 (Athey et al., 2020[23]). To mitigate the massive losses to the global economy due to COVID‑19, the model shows that it would be optimal for governments to collectively invest around USD 137 billion to accelerate vaccine development and manufacturing, through building capacity in parallel with development. Participating countries could contribute in proportion to their GDP. Funds would be allocated through a combination of push and pull mechanisms. Different scenarios also show that a large share of the welfare gain can be achieved with a smaller investment – for example a total budget of USD 75 billion could achieve more than 90% of the welfare gain. The parameters of this model can thus be adapted. To estimate the funding required, the model also requires estimates of success probabilities of vaccine candidates in R&D and assumes that there is some correlation between success probabilities of the various candidates. These inputs need refinement and will change over time as the R&D pipeline evolves. However, the base case estimate shows the order of magnitude of funding required.
If implemented successfully, the scheme would result in a monthly manufacturing capacity of at least 500 million doses, effectively allowing to vaccinate nearly the entire global population within one year. By reducing product scarcity, capacity at this massive scale could avoid costly bidding wars between countries and pre-empt disputes over trade restrictions or licensing of intellectual property rights (IPRs).
In the baseline scenario of the model, USD 45 billion of the total funding would be committed in a global AMC to incentivise developers of 18 vaccine candidates to join the scheme and have an 80% probability that a least 1 of the 18 would be effective and manufactured within 18 months. With the remaining budget, governments would directly fund 85% of the cost of building manufacturing capacity for all 18 candidates in development, estimated to cost USD 6 billion per candidate. Firms bear the remaining 15% of the capacity cost and, in case their candidate is successful, the full marginal production cost. Within 3 months of marketing authorisation, 1.5 billion doses would be delivered to high-risk groups (including health care workers and people with comorbidities), followed by 1.5 billion doses to the wider population within another 3 months. To incentivise speed and provide attractive returns to the first successful candidate(s), the first 1 billion doses would be purchased at a price of USD 35 per dose and the remaining 2 billion at USD 5 per dose.
The Centre for Global Development also proposed an AMC design to incentivise R&D and scale-up of production capacity (Silverman et al., 2020[16]). The proposal also accounts for differentials in countries’ ability to pay in pricing. Prices are tiered across countries based on levels of wealth and health care budgets. The AMC would disburse funds to several successive vaccines to keep several potential innovators in the game, conditional on licensing the IPRs to local producers at a low or zero cost to help widespread uptake of the vaccine.
Manufacturing capacity has to be built while R&D is underway
Once a vaccine that is safe and effective has been developed, it needs to be manufactured at scale. Although it is difficult at this stage to estimate with any precision the number of doses that will have to be produced and delivered to populations over a short period of time, billions of doses will likely be necessary and their manufacture and distribution will be a formidable task. It is of course still unknown how many doses of a future vaccine will have to be administered for the human body to build immunity. Multiple doses may be needed for the same individual, should the vaccine require boosts to be effective.
Little information about vaccine manufacturing capacity is publicly available. However, experience from prior efforts to monitor capacity to manufacture influenza vaccines indicates that production cannot be scaled up rapidly. Vaccine manufacturing tends to be technically more complicated than producing medicines and requires a broad range of technology, human resources and quality controls (Smith, Lipsitch and Almond, 2011[23]; UNIDO and WHO, 2017[24]). Building manufacturing lines requires sustained investment and has, in the past, taken several years (UNIDO and WHO, 2017[24]). In 2015, there was global capacity to manufacture approximately 6 billion doses of influenza vaccines in case of a pandemic, sufficient for immunising about 40% of the global population with two doses (McLean et al., 2016[25]). But this capacity is only available because the same manufacturing sites produce already some 1.5 billion doses of seasonal influenza vaccinations every year (ibid.). This illustrates that, even with well-prepared capacity, it takes time to scale up production.
Countries therefore need to co-operate now to project demand and plan the necessary capacity to produce doses in sufficient quantities. While attention has so far focused on spurring R&D, there has been little co-ordination or co-operation between countries in funding, planning and building manufacturing capacity. Because it takes time to build capacity, this effort has to start now, while R&D is still underway. CEPI is already considering manufacturing capacity in its projections of funding needs.10 Several large pharmaceutical firms have recently announced that they are starting to build manufacturing capacity for future vaccine candidates, some with backing by public funders.
Capacity will have to be funded, at least partly, from public sources because uncertainty is still too high to rely only on private investment. As discussed in Box 3, an international AMC that is large enough could provide incentives for the private sector to invest not only in R&D but also in manufacturing capacity, to complement direct public funding of capacity. Alternatively, countries could also co-operate to fund the entire needed capacity directly, and separate from incentives for R&D. One source of uncertainty is that it is still unknown which of the vaccine platforms currently in trials, discussed above, will turn out to be successful. Different manufacturing challenges are associated with each vaccine platform and production lines cannot manufacture all platforms (Smith, Lipsitch and Almond, 2011[23]). If a future vaccine is based on a platform that is already used for other authorised vaccines, existing capacity can be repurposed and expanded. However, this may, in turn, create capacity bottlenecks for vaccines against other diseases. Completely new production infrastructure, however, will have to be built for new vaccine platforms, such as DNA and mRNA vaccines. Another source of uncertainty is that it is not known when a vaccine will be authorised for marketing and how big the market will be at that point. The longer it takes to complete R&D, the smaller the market for a vaccine may become, as more and more people get infected and possibly build immunity and as societies adapt to living with an endemic virus.
Progress towards treatments is mainly being made by repurposing existing medicines
Treatments tested so far have provided disappointing results
Most of the clinical trials underway by May 2020 are testing treatments. Rather than coming up with compounds from scratch that may take years to develop and test, researchers are currently mainly looking to repurpose medicines already approved for other diseases and known to already have a positive benefit/risk balance for conditions other than COVID‑19. They are also looking at unapproved drug candidates that have performed well in pre-clinical studies with the past two deadly outbreaks of coronaviruses between in 2002 and 2012, which caused severe acute respiratory syndrome (SARS) and Middle East respiratory syndrome (MERS) respectively.
Given the current state of knowledge and limited progress so far, expectations that treatments will become game changers in the fight against SARS‑CoV‑2 need to be tempered. The drug candidates currently being tested have potential to improve the clinical management of patients with COVID‑19 but do not provide a cure and are unlikely to be used to prevent people from getting sick. As of 26 May, there is no evidence from randomised controlled trials (RCTs) that any potential therapy under investigation improves outcomes in patients with either suspected or confirmed COVID‑19. Most of them are associated with significant side effects and target narrow patient groups, such as the most severe cases. Also, some of them can only be administered intravenously, limiting their use to hospital settings.
With more than 500 clinical trials of treatments currently underway (Thorlund et al., 2020[27]), including the large-scale trials “Solidarity” and “Discovery”,11 the state of knowledge will evolve rapidly. International co-ordination is needed to ensure that clinical trials are well-designed, adhere to common methodological standards and are sufficiently large to yield conclusive information. This has not always been the case across the hundreds of trials in the COVID‑19 R&D effort so far, which included many disparate and small-scale trials of the same treatments (Mullard, 2020[28]; Thorlund et al., 2020[27]). The controversies around hydroxychloroquine and chloroquine and the current uncertainty around the effects of remdesivir are examples of how small-scale trials can cause confusion (Nature, 2020[29]). Some innovative approaches are emerging to ensure high standards of research in the context of the epidemic. For example, the REMAP-CAP study tests a variety of treatments against COVID‑19. It will include participants from more than 160 sites across 14 countries. Sophisticated clinical trial designs allow researchers to add treatments to the trial as it is running, and to remove those for which data indicate that they are not performing well. REMAP-CAP was originally designed to study pneumonia and has now refocused on COVID‑19 (ibid.).
Table 3 contains an overview of COVID‑19 treatments being assessed as of 26 May that have attracted the most attention and are being considered the most promising. The different strategies being tested try to tackle the infection at three different steps of its natural history:
Prevent the virus from getting into the host’s cells; this is the case of chloroquine/hydroxychloroquine and antibody-based treatments made from convalescent plasma;
Prevent the virus from replicating once inside the host’s cell; this is how most antivirals act;
Prevent the over-reaction of the immune system to the infection (the so-called “cytokine storm”, which is responsible of the severe cases of COVID‑19 infections); this is the case of some immunosuppressive treatments
Eventually, the treatments currently tested may contribute to building some sort of a “tool box”, from which doctors would choose to manage patients on a case by case basis. Emergency measures such as the Emergency Use Authorization (EUA) of the US Food and Drug Administration (FDA) may contribute to accelerating approvals but phase 3 double-blind randomised controlled trials (the gold standard in medical investigation) take several months. Despite the urgency, exceptional circumstances should not be a reason for authorising treatments that cause more harm than benefit (London and Kimmelman, 2020[8]).
Table 3. Overview of main COVID‑19 treatments currently being assessed
Status per 26 May 2020
|
Therapeutic |
Approval status and availability of generics |
Patient target group per clinical trials underway (moderate, severe, critical stage of the disease) |
Route of administration |
---|---|---|---|---|
Preventing virus from entering host’s cell |
Chloroquine or Hydroxychloroquine1 |
Approved for malaria, autoimmune diseases. FDA Emergency Use Authorisation for COVID‑19 (hospitalised patients only). Generic(s) available. |
Moderate, severe (not yet established) |
Oral |
Umifenovir |
Approved for influenza in Russia/China. Not approved for any indication in United States or EU. |
Not established yet |
Oral |
|
COVID‑19 convalescent plasma |
Not (FDA/EMA) approved but can be used in investigational capacity. |
Severe, critical |
Intravenous |
|
Blocking viral replication in host’s cell |
Remdesivir |
Not formally approved for any disease yet. Available under FDA Emergency Use Authorisation for COVID‑19 in the US market. EMA has issued recommendations for compassionate use in Europe. |
Severe, critical |
Intravenous |
Ritonavir/lopinavir |
Approved for HIV. Generic(s) available. |
Not formally established yet (moderate to severe) |
Oral or intravenous |
|
Ribavirin |
Approved for hepatitis C. Generic(s) available. |
Not formally established yet (moderate to severe) |
Oral |
|
Oseltamivir |
Approved in influenza. Generic(s) not available. |
Not formally established yet (moderate to severe) |
Oral |
|
Favipiravir |
Approved in Japan for influenza. Not approved for any indication in United States. Available only for compassionate use in COVID‑19 in United States. |
Not formally established yet (moderate to severe) |
Oral |
|
Intravenous route of addressing over-reaction of the immune system |
Tocilizumab |
Approved in diseases other than COVID‑19 (e.g. rheumatoid arthritis, cytokine release syndrome). Generic(s) not available. |
Severe, critical |
Intravenous |
Sarilumab |
Approved in rheumatoid arthritis. Generic(s) not available. |
Severe, critical |
Subcutaneous |
Note: The status of the development pipeline is evolving rapidly. Information in this table may be out-of-date quickly.
1. On 25 May 2020, WHO suspended the utilisation of chloroquine / hydroxychloroquine in its Solidarity trial based on the most recent evidence published, which highlighted the unfavourable benefit/risk balance of these two molecules in the context of COVID‑19.
Policy also needs to ensure that effective treatments will be widely available
Medicines go through stages between discovery and delivery to patients that are similar to vaccines, as illustrated in Figure 1. However, there are some important differences. Most importantly, medicines are only given to people who are already infected with SARS‑CoV‑2 and usually target specified patient groups, such as severe and critical cases. The product volume to be manufactured may therefore be smaller than in the case of vaccines. While it is difficult to find chemical compounds that are effective in treating a disease, medicines made of chemical ingredients are relatively cheap and straightforward to manufacture.
Nevertheless, pharmaceutical supply chains may not be prepared for supplying sufficient volumes of treatments during a pandemic. Even if some medicines are relatively straightforward to manufacture, their production frequently relies on rare chemical ingredients that can only be procured from a small number of suppliers. Supply chains can be complex and it is enough that one critical input is in short supply for production to grind to a halt (Ledford, 2020[30]). The high and increasing number of medicine shortages reported in recent years in OECD countries12 is an indicator of an already tense situation in this industrial sector and health emergencies, such as the ongoing pandemic, adds to the risk of shortages (Ledford, 2020[31]).
Policy also needs to ensure that there will be sufficient capacity to manufacture effective medicines at scale and that products will be widely accessible. This includes providing the right incentives to manufacturers and building the necessary capacity in global supply chains. Most importantly, policy needs to ensure that medicines will be accessible to those in need and that pricing and barriers to international trade do not constitute barriers to access. This is discussed in the next section.
Rules for intellectual property rights, affordable pricing and procurement should be agreed upfront to ensure efficient and equitable access
Equal and broad access to any new vaccine for SARS‑CoV‑2 and to medicines for treating COVID‑19 across all countries is key if these products are to allow societies to live with the virus, restore international travel, trade and business confidence, and eventually return to ‘normal’ life. This requires that the current regime of intellectual property rights (IPRs) is applied in a way to ensure that research is not hampered, that new knowledge is widely disseminated, and that product prices do not constitute access barriers, while providing sufficient and adequate returns to manufacturers. It also requires that countries co-ordinate procurement and distribution of products.
Intellectual property rights incentivise R&D and there are various ways to ensure affordability of health technologies
In the current rush for finding a way out of the public health crisis, financial incentives for R&D need to be reconciled with affordability and equity concerns. Usually, pharmaceutical R&D mainly relies on various forms of intellectual property rights (IPRs) to incentivise private investment. Patents, in particular, are critical for incentivising R&D. They also favour the dissemination of new knowledge because they require disclosure.13 While critical for incentivising R&D, IPRs give their owners market power to limit supply and obtain high prices, which can create barriers to broad access to health technologies. In the context of COVID‑19, vast amounts of public funding have already allocated to R&D and, as argued above, more funding will be needed. Given that taxpayers already bear much of the risk and costs of R&D and that broad access to a new vaccine and effective treatments will be key to restoring social and economic life, IPRs should not create financial access barriers and product prices will need to be close to the cost of production to ensure affordability. How countries share the cost of R&D and production will mainly depend on the contributions respective governments make to funding push and pull mechanisms for R&D and manufacturing.
The current regime of intellectual property rights will apply to a future vaccine and treatments
All vaccines in development are new and will likely be patentable regardless of who develops them – the public or private sectors or public-private partnerships. Most medicines in clinical trials to treat COVID‑19 are already authorised for other diseases (they include off- and on-patent products). But repurposed medicines can also be subject to intellectual property protection in some jurisdictions, including in Europe, the United States and some other countries, and under certain conditions (see Ducimetière (2019[26])).
Independent from patents, new medicines are also shielded from generic competition by data and market exclusivity in many OECD countries, including Australia, Canada, Japan, the United States and EU member states. Data exclusivity prevents generic manufacturers, for a certain period of time from marketing authorisation of the originator, from referring to data from pre-clinical studies and clinical trials of the originator to demonstrate safety and efficacy of the generic. During periods of market exclusivity, generic manufacturers can prepare and apply for marketing authorisation but are not permitted to market their products to compete with the originator. These exclusive rights are granted by regulatory agencies (’t Hoen, Boulet and Baker, 2017[34]). Even in the absence of patent protection, they effectively preclude generic market entry for a period of between 5 to 12 years from marketing authorisation of the originator, depending on the jurisdiction and type of medicine (Boulet, Garrison and ‘t Hoen, 2019[35]; ’t Hoen, Boulet and Baker, 2017[34]). In the absence of licenses that waive such rights, they can impede the quick scaling of production of new medicines during a pandemic.
Governments can use various tools to ensure that intellectual property rights do not create barriers to access
Various options are emerging to manage IPRs during this crisis, for instance, by combining “open innovation” models and patent pools. These accelerate technological progress by encouraging universities and firms venturing in early stages of R&D to make their IPR available to firms investing in follow-on clinical studies and product development, while maintaining incentives for firms to compete using their exclusive IPR. Another option is to tie public R&D funding, whether allocated through push or pull mechanisms, to conditions for accessibility and affordability. Entities that fund R&D can, for instance, demand upfront that products resulting from the R&D they fund be subject to voluntary licenses (VLs) or to be placed in the public domain. Governments can also acquire IPRs or, where appropriate, use flexibilities provided in the current IPR regime to contain prices.
“Open innovation” initiatives have been recently adopted by universities in the United States and others. On 7 April, Harvard University, the Massachusetts Institute of Technology, and Stanford University have committed to a set of technology licensing principles that will incentivise and allow for the most broad and equitable access to their innovations. The principles provide for non-exclusive, royalty-free licensing of IPRs to most types of technologies during the COVID‑19 pandemic and for a short period afterwards. Licensees will be expected to distribute the resulting products as widely as possible and at a low cost that allows broad accessibility.14
Patent pools are arrangements under which two or more holders of patents in a technology license them as a bundle to third parties. Pools are particularly helpful when they reduce transaction costs by licensing of technologies that require the simultaneous use of several patented technologies, although there are concerns about their reducing competition between rival technologies (WIPO, 2014[27]; Lerner and Tirole, 2002[28]). The rules governing patent pools can vary considerably, including in terms of how members share profits, whether non-members of the pool can be licensees, and whether patents can also be licensed individually (Lerner and Tirole, 2002[28]).
The government of Costa Rica and advocacy groups are calling for a global shared pool of rights for COVID-related IP with “free access or licensing on reasonable and affordable terms”.15 Some other initiatives are already underway. The Open Covid Pledge of the University of Utah was set up in collaboration between scientists, inventors, lawyers and the technology industry to facilitate access to basic licensing agreements for coronavirus-related technologies.16 The Medicine Patent Pool (MPP) designed to facilitate license agreements between patent holders and licensees, namely generic manufacturers meeting quality standard requirements, has also extended its mandate to be able to play such a role for COVID‑19 related vaccines or medicines.17 All MPP licences include a data exclusivity waiver to facilitate marketing authorisation of medicines manufactured by licensees (’t Hoen, Boulet and Baker, 2017[34]).
Voluntary licenses (VLs) are a mechanism for patent holders to voluntarily allow other parties to exploit their patents. VLs have been applied to several discoveries in biotechnology, genetics and, lately, to patented products repurposed for COVID‑19.18 VLs are particularly beneficial when innovations have a high degree of cumulativeness, i.e. when a subsequent innovation depends on a previous one. But VLs may face a number of obstacles, the main being disagreement between the parties about the value of the innovation to be licensed and royalties to be paid (Scotchmer, 2004[29]).
Another option is linking pull mechanisms to conditions for IPR licensing and affordability and access. The Advance Market Commitment (AMC) proposal described above would entail a condition for disbursement of funds related to the licensing of IPR to local producers at a low or even no or zero cost to help broad production and widespread uptake of the vaccine.
Rather than relying on VLs or making pull mechanisms, including AMCs to purchase a specified volume of a product at a pre-determined prices, on licensing commitments, governments could also commit to acquiring IPR in new technologies. This would also incentivise R&D and could allow governments to put the ensuing IPR in the public domain, effectively making the knowledge a global public good.
Finally, governments can prepare or activate mechanisms in national legislation that allow for flexibilities to apply to patent protection, including compulsory licensing, especially in cases where VLs are refused or too slow to respond to an urgent situation. Some countries have already taken action in response to the COVID‑19 pandemic, including Canada, Chile and Germany and Israel.19 However, because compulsory licenses (CLs) can be issued unilaterally, their wider use risks undermining the incentives that IPRs create and can have undesired effects on R&D (OECD, 2018[28]). The pull mechanisms discussed above, including a possible international advance market commitment, could provide an alternative to IPR-based incentives for R&D and help avoid the use of CLs.
The use of flexibilities to patent protection is recognised under international treaties: the World Trade Organization (WTO) Agreement on Trade-Related Aspects of Intellectual Property Rights (TRIPS) leaves significant leeway to governments in applying such flexibilities to achieve national policy objectives, including, among other reasons, where these are necessary to protect public health. Flexibilities allow, among other things, for making exceptions to existing patent protection (Article 30) and compulsory licencing (Article 31).20 A compulsory license (CL) allows a firm who does not hold the patent to produce the patented product or use the patented production process without authorisation of the patent owner. Compulsory licensing, in principle, entails a requirement to remunerate the patent owner adequately and usually has to be preceded by an attempt to obtain authorisation from the owner on reasonable commercial terms. But the latter requirement does not apply if use of the licence is for public and non-commercial use or in response to a national emergency or other circumstances of extreme urgency (Article 31). The 2001 Doha Declaration on the TRIPS Agreement and Public Health reaffirmed that, “the Agreement can and should be interpreted and implemented in a manner support of WTO Members’ right to protect public health and, in particular, to promote access to medicines for all.” 21 It clarified the right of countries to grant CLs, as well as the freedom to determine the grounds for such licenses and also to determine what constitutes a national emergency or other circumstances of extreme urgency. Health technologies produced under normal CLs, which are available to all WTO Members for all patented products and processes, must be predominantly provided to the domestic market. A subsequent amendment to the TRIPS Agreement (Article 31bis) allows low cost generic medicines to be produced and exported under a CL exclusively for the purpose of serving the needs of countries that cannot manufacture those products themselves.22 It applies to pharmaceutical products, including medicines, vaccines and diagnostics, needed to fight an epidemic. Eligible importers include developing and least developed countries.
Governments that aim to use CLs also have to ensure that licensees are not precluded from marketing their products as a result of data or market exclusivity (see above). This can be challenging because these exclusive rights are separate from patents and not all jurisdictions provide mechanisms to automatically waive them if a CL is issued. A CL may therefore have no effect on the validity of data and market exclusivity rights that prevent generics or biosimilars from entering the market. European Union law, for example, only provides waivers to data and market exclusivity in case of compulsory licensing for the manufacture of pharmaceutical products for export but not for products marketed inside the EU (Boulet, Garrison and ‘t Hoen, 2019[35]; ’t Hoen, Boulet and Baker, 2017[34]).
Countries need to agree how to distribute new vaccines to avoid future trade disputes and bidding wars
Upfront agreements among countries on how to allocate scarce product volumes are necessary. This is to avoid potential unilateral action by some countries, including panic-buying and excessive stockpiling and export bans by producing countries. Such behaviour has occurred in the past, for instance in the 2009 influenza pandemic.23
Procurement schemes based upon international co-operation need to be defined upfront and have to identify a criterion for allocating product volumes to countries that is not based on the ability to pay. Countries could, for example, agree that those hit hardest by the pandemic will have priority access to vaccines. Volumes could also be allocated according to population size, with each country receiving a share of vaccines that is proportionate to its share of the total population of the countries that co-operate, or according to the size of population groups that are particularly high-risk, such as the elderly and people with chronic diseases. Given the global scale of the pandemic, co-operation would have to be broad, involving as many countries as possible.
An agreement could also comprise a broader commitment to trade in medical supplies. Multilateral discussions on facilitating cross-border flows of vital medical supplies and other essential goods and services are already underway, including proposals on eliminating tariffs on these products. For instance, on 5 May, 44 WTO Members circulated a statement on COVID‑19 and the multilateral trading system committing to “encourage work at the WTO on concrete actions aimed at facilitating cross-border flows of vital medical supplies and other essential goods and services, including through the application of best practices and simplified procedures and through further trade opening.”24 Concrete proposals have been made by New Zealand and Singapore for a “Declaration on Trade in Essential Goods for Combating the COVID‑19 Pandemic” (WTO, 2020 G/C/W/777).25 It provides a list of critical goods and proposes, among other things, tariff elimination, restrictions on export prohibitions, intensified consultations on non-tariff barriers, and greater facilitation of trade.
Experiences from existing international joint procurement schemes can provide lessons to countries on how to plan demand for a vaccine ex-ante and put co-operation into practice. Procurement comprises the entire process of acquisition of supplies at specified prices, and at recognised standards of quality, involving a wide range of activities including price negotiations, demand forecasting, budgeting, and timely delivery (Management Sciences for Health, 2012[30]). Prior examples of international co-operation in procurement range from loose arrangements of information sharing to more integrated forms, where participating countries may open joint tenders, enter into contracts jointly or set up a central buying unit that enters into economic transactions with suppliers (World Health Organization, 2016[31]).
For instance, the Pan American Health Organization (PAHO) Revolving Fund for Vaccine Procurement established in 1979 is a joint procurement scheme that now comprises 41 Latin American and Caribbean countries (World Health Organization, 2016[31]; DeRoeck et al., 2006[32]). The Revolving Fund represents a high level of integration, with tendering, contracting and payment performed centrally by PAHO. A more recent example comes from Europe. The EU is now making use of the Joint Procurement Mechanism26 established in the aftermath of the H1N1 influenza pandemic to deal with the COVID‑19 crisis. This sets a joint procurement procedure to purchase vaccines, antivirals and other medical supplies, including medical devices and other goods or services aimed “at combating serious cross-border threats to health” (European Commission, 2016[33]). As of April 2020, 36 countries have signed this, including all EU and EEA countries, as well as the United Kingdom, Albania, Montenegro, North Macedonia, Serbia and Bosnia and Herzegovina. This mechanism represents a high degree of integration between participating member countries in that the European Commission takes central responsibility for the procurement process, opens tenders on behalf of countries, becomes the sole contractual counter party for suppliers and allocates products to member countries (ibid.). However, there is no requirement for exclusivity and member states can continue to contract individually with suppliers while participating in the scheme; they are also allowed to opt out of the scheme at any stage (Azzopardi-Muscat, Schroder-Beck and Brand, 2016[34]).
References
[4] Amanat Fatima, K. (2020), “SARS-CoV-2 vaccines: status report”, Cell, Vol. 52/4, pp. 1-7, http://dx.doi.org/10.1016/j.immuni.2020.03.007.
[23] Athey, S. et al. (2020), Incentive Design to Accelerate a COVID-19 Vaccine, May 23.
[44] Azzopardi-Muscat, N., P. Schroder-Beck and H. Brand (2016), “The European Union Joint Procurement Agreement for cross-border health threats: what is the potential for this new mechanism of health system collaboration?”, Health Economics, Policy and Law, pp. 1-17, http://dx.doi.org/10.1017/S1744133116000219.
[35] Boulet, P., C. Garrison and E. ‘t Hoen (2019), Data Exclusivity in the European Union: Briefing Document, Medicines Law & Policy, https://medicineslawandpolicy.org/wp-content/uploads/2019/06/European-Union-Review-of-Pharma-Incentives-Data-Exclusivity.pdf (accessed on 13 May 2020).
[21] Chalkidou, K. et al. (2020), Blueprint for a Market-Driven Value-Based Advance Commitment for Tuberculosis, Centre for Global Development, Washington, https://www.cgdev.org/sites/default/files/MVAC-Blueprint-Final_2.pdf (accessed on 28 April 2020).
[11] Chen, W. et al. (2020), “The SARS-CoV-2 Vaccine Pipeline: an Overview”, Current Tropical Medicine Reports, pp. 1-4, http://dx.doi.org/10.1007/s40475-020-00201-6.
[42] DeRoeck, D. et al. (2006), “Regional group purchasing of vaccines: Review of the Pan American Health Organization EPI revolving fund and the Gulf Cooperation Council group purchasing program”, International Journal of Health Planning and Management, Vol. 21/1, pp. 23-43, http://dx.doi.org/10.1002/hpm.822.
[33] Ducimetière, C. (2019), “Second Medical Use Patents-Legal Treatment and Public Health Issues”, http://www.southcentre.int (accessed on 15 April 2020).
[2] EMA (2020), EU Clinical Trials Register, https://www.clinicaltrialsregister.eu/.
[43] European Commission (2016), Joint procurement of medical countermeasures, http://ec.europa.eu/health/preparedness_response/joint_procurement/index_en.htm.
[10] Flaxman, S. et al. (2020), Estimating the number of infections and the impact of non-pharmaceutical interventions on COVID-19 in 11 European countries, MRC Centre for Global Infectious Disease Analysis, London, https://www.imperial.ac.uk/media/imperial-college/medicine/sph/ide/gida-fellowships/Imperial-College-COVID19-Europe-estimates-and-NPI-impact-30-03-2020.pdf (accessed on 31 March 2020).
[22] GAVI AMC Secretariat (2019), Advance Market Commitment For Pneumococcal Vaccines: Annual Report 2018, GAVI, https://www.gavi.org/sites/default/files/document/2018-pneumococcal-amc-annual-reportpdf.pdf (accessed on 5 May 2020).
[14] Gouglas, D. et al. (2018), “Estimating the cost of vaccine development against epidemic infectious diseases: a cost minimisation study”, The Lancet Global Health, Vol. 6/12, pp. e1386-e1396, http://dx.doi.org/10.1016/S2214-109X(18)30346-2.
[7] Iwasaki, A. and Y. Yang (2020), “The potential danger of suboptimal antibody responses in COVID-19.”, Nature reviews. Immunology, pp. 1-3, http://dx.doi.org/10.1038/s41577-020-0321-6.
[6] Jiang, S. (2020), “Don’t rush to deploy COVID-19 vaccines and drugs without sufficient safety guarantees”, Nature, Vol. 579/7799, pp. 321-321, http://dx.doi.org/10.1038/d41586-020-00751-9.
[13] Kremer, M. (2004), Strong Medicine: Creating Incentives for Pharmaceutical Research on Neglected Diseases, Princeton University Press, Princeton, New Jersey.
[32] Ledford, H. (2020), “Dozens of coronavirus drugs are in development — what happens next?”, Nature, http://dx.doi.org/10.1038/d41586-020-01367-9.
[37] Lerner, J. and J. Tirole (2002), Efficient Patent Pools, National Bureau of Economic Research Working Paper 9175, Cambridge, MA, http://dx.doi.org/10.3386/w9175.
[20] Levine, R., M. Kremer and A. Albright (2005), Making Markets for Vaccines: Ideas to action. The report of the Center for Global Development Advance Market Commitment Working Group, Center for Global Development, Washington D.C., https://www.cgdev.org/sites/default/files/archive/doc/books/vaccine/MakingMarkets-complete.pdf.
[31] London, A. and J. Kimmelman (2020), “Against pandemic research exceptionalism”, Science, Vol. 368/6490, p. eabc1731, http://dx.doi.org/10.1126/science.abc1731.
[1] LSHTM Vaccine Centre (2020), COVID-19 vaccine development pipeline, https://vac-lshtm.shinyapps.io/ncov_vaccine_landscape/ (accessed on 10 April 2020).
[40] Management Sciences for Health (2012), MDS-3: Managing Access to Medicines and Health Technologies, Management Sciences for Health, Arlington, VA, USA, http://www.msh.org/resource-center/ebookstore/copyright.cfm.%5Cnwww.mds-online.org.
[27] McLean, K. et al. (2016), “The 2015 global production capacity of seasonal and pandemic influenza vaccine”, Vaccine, Vol. 34/45, pp. 5410-5413, http://dx.doi.org/10.1016/j.vaccine.2016.08.019.
[12] Menachery, V. et al. (2015), “A SARS-like cluster of circulating bat coronaviruses shows potential for human emergence”, Nature Medicine, Vol. 21/12, pp. 1508-1513, http://dx.doi.org/10.1038/nm.3985.
[18] Morel, C. and E. Mossialos (2010), Stoking the antibiotic pipeline, British Medical Journal Publishing Group, http://dx.doi.org/10.1136/bmj.c2115.
[19] Mossialos, E. et al. (2010), Policies and incentives for promoting innovation in antibiotic research, European Observatory on Health Systems and Policies, https://apps.who.int/iris/bitstream/handle/10665/326376/9789289042130-eng.pdf (accessed on 5 May 2020).
[29] Mullard, A. (2020), “Flooded by the torrent: the COVID-19 drug pipeline”, The Lancet, Vol. 395/10232, pp. 1245-1246, http://dx.doi.org/10.1016/S0140-6736(20)30894-1.
[46] Musmann, T. (2020), “German Government Plans Possibilities to Limit Patents In View of Corona Pandemic”, Kluwer Patent Blog, http://patentblog.kluweriplaw.com/2020/03/24/german-government-plans-possibilities-to-limit-patents-in-view-of-corona-pandemic/.
[30] Nature (2020), “Coronavirus drugs trials must get bigger and more collaborative”, Nature, Vol. 581/7807, pp. 120-120, http://dx.doi.org/10.1038/d41586-020-01391-9.
[39] OECD (2018), Pharmaceutical Innovation and Access to Medicines, OECD Health Policy Studies, OECD Publishing, Paris, https://dx.doi.org/10.1787/9789264307391-en.
[8] Pronker, E. et al. (2013), “Risk in Vaccine Research and Development Quantified”, PLoS ONE, Vol. 8/3, p. 57755, http://dx.doi.org/10.1371/journal.pone.0057755.
[45] Sanche, S. et al. (2020), “High Contagiousness and Rapid Spread of Severe Acute Respiratory Syndrome Coronavirus 2.”, Emerging infectious diseases, Vol. 26/7, http://dx.doi.org/10.3201/eid2607.200282.
[38] Scotchmer, S. (2004), Innovation and incentives, MIT Press.
[24] Silverman, R. et al. (2020), FINANCING AND SCALING INNOVATION FOR THE COVID FIGHT Financing and Scaling Innovation for the COVID Fight: A Closer Look at Demand-Side Incentives for a Vaccine, Center for Global Development, Washington D.C., https://www.cgdev.org/sites/default/files/Silverman-Krubiner-Chalkidou-Towse-R%26D-COVID.pdf (accessed on 2 April 2020).
[17] Simpson, S. et al. (2020), Disease X: accelerating the development of medical countermeasures for the next pandemic, Lancet Publishing Group, http://dx.doi.org/10.1016/S1473-3099(20)30123-7.
[25] Smith, J., M. Lipsitch and J. Almond (2011), “Vaccine production, distribution, access, and uptake”, The Lancet, Vol. 378/9789, pp. 428-438, http://dx.doi.org/10.1016/S0140-6736(11)60478-9.
[47] ’t Hoen, E. (2020), “Covid-19 and the comeback of compulsory licensing”, Medicines Law & Policy, https://medicineslawandpolicy.org/2020/03/covid-19-and-the-come-back-of-compulsory-licensing/.
[34] ’t Hoen, E., P. Boulet and B. Baker (2017), “Data exclusivity exceptions and compulsory licensing to promote generic medicines in the European Union: A proposal for greater coherence in European pharmaceutical legislation”, Journal of Pharmaceutical Policy and Practice, Vol. 10/1, p. 19, http://dx.doi.org/10.1186/s40545-017-0107-9.
[5] Thanh Le, T. et al. (2020), “The COVID-19 vaccine development landscape”, Nature Reviews Drug Discovery, http://dx.doi.org/10.1038/d41573-020-00073-5.
[28] Thorlund, K. et al. (2020), “A real-time dashboard of clinical trials for COVID-19”, The Lancet, http://dx.doi.org/10.1016/S2589-7500(20)30086-8.
[26] UNIDO and WHO (2017), Establishing Manufacturing Capabilities for Human Vaccines, https://www.unido.org/sites/default/files/files/2017-12/Establishing-Manufacturing-Capabilities-for-Human-Vaccines-ebook.pdf (accessed on 27 April 2020).
[3] US NIH (2020), ClinicalTrials.gov, https://clinicaltrials.gov.
[15] Widdus, R. (2001), “Public-private partnerships for health: their main targets, their diversity, and their future directions”, Bulletin of the World Health Organization, Vol. 79/8, pp. 713-720, http://dx.doi.org/10.1590/S0042-96862001000800006.
[16] Widdus, R. et al. (2001), Combating Diseases Associated with Poverty Financing Strategies for Product Development and the Potential Role of Public-Private Partnerships IPPPH SECRETARIAT.
[36] WIPO (2014), Patent Pools and Antitrust – A Comparative Analysis, World Intellectual Property Organization (WIPO), https://www.wipo.int/export/sites/www/ip-competition/en/studies/patent_pools_report.pdf (accessed on 29 April 2020).
[9] Wong, C., K. Siah and A. Lo (2018), “Estimation of clinical trial success rates and related parameters”, Biostatistics February, pp. 1-14, http://dx.doi.org/10.1093/biostatistics/kxx069.
[41] World Health Organization (2016), Challenges and opportunities in improving access to medicines through efficient public procurement in the WHO European Region.
Contact
Stefano SCARPETTA (✉ stefano.scarpetta@oecd.org)
Mark PEARSON (✉ mark.pearson@oecd.org)
Francesca COLOMBO (✉ francesca.colombo@oecd.org)
Eliana BARRENHO (✉ eliana.barrenho@oecd.org)
Guillaume DEDET (✉ guillaume.dedet@oecd.org)
Martin WENZL (✉ martin.wenzl@oecd.org)
Notes
← 1. Clinical trials of medicines and vaccines usually occurs in 3 sequential phases: phase 1 trials usually assess safety and tolerability in a small group of less than 100 adults; phase 2 trials test safety, dosage and method of delivery in a larger group of several hundred people; and, phase 3 trials aim to establish safety and efficacy in a large group of several hundred to thousands of people.
← 2. That is, 2 to 4 new infections generated by every existing infection in a population that is not immune. Estimates of R0 currently vary widely, but range up to 6 (Sanche et al., 2020[35]).
← 3. See OECD (2020), Beyond Containment: Health systems responses to COVID‑19 in the OECD. Available at https://www.oecd.org/coronavirus/en/.
← 9. See GAVI’S Proposal for an Advance Market Commitment for COVID‑19 Vaccines, at https://www.gavi.org/sites/default/files/covid/Gavi-proposal-AMC-COVID-19-vaccines.pdf.
← 10. See https://cepi.net/news_cepi/2-billion-required-to-develop-a-vaccine-against-the-COVID-19-virus-2/.
← 11. The global Solidarity trial overseen by the WHO and the European Discovery trial overseen by the French National Institute of Health and Medical Research (INSERM). Both trials were initiated in March 2020 and will follow patients for several years. See https://www.who.int/emergencies/diseases/novel-coronavirus-2019/global-research-on-novel-coronavirus-2019-ncov/solidarity-clinical-trial-for-COVID-19-treatments and https://presse.inserm.fr/en/launch-of-a-european-clinical-trial-against-COVID-19/38737/.
← 12. An OECD report on medicine shortages will be published in mid‑2020.
← 13. Various national authorities as well as the World Intellectual Property Organization (WIPO) have aimed to facilitate access to information in patent applications relevant to COVID‑19. WIPO established the Patentscope COVID‑19 Index, see https://patentscope.wipo.int/search/en/covid19.jsf.
← 15. See https://healthgap.org/rationale-for-supporting-costa-ricas-proposal-for-emergency-COVID-19-technology-ip-pool-for-all-countries/ and https://www.keionline.org/32556.
← 17. https://medicinespatentpool.org/mpp-media-post/the-medicines-patent-pool-and-unitaid-respond-to-access-efforts-for-COVID-19-treatments-and-technologies/.
← 18. For example, AbbVie voluntarily waived any restriction on the MPP licensees that would prevent generic companies from supplying lopinavir/ritonavir (Kaletra®), including restrictions on the generic supply of this treatment for HIV (see ’t Hoen (2020[47])). Gilead announced plans to issue VLs for remdesivir, see https://www.statnews.com/pharmalot/2020/05/05/gilead-remdesivir-covid19-coronavirus/.
← 19. Bill C‑13 in Canada’s facilitates the grant of government use licences; in Chile, a resolution by Parliament called upon government to grant CLs; Germany declared an epidemic of national importance and empowers the Ministry of Health to order the grant of a CL; Israel issued a normal CL under Article 31 TRIPS for the supply of lopinavir/ritonavir (Kaletra®) while the patent holder waived enforcement of patent rights (see note 18 above). See, for example, Musmann (2020[43]) and ’t Hoen (2020[47]).
← 20. Article 31 of the TRIPS Agreement does not mention the term “compulsory licenses” explicitly but refers to “use without the authorisation of the patent owner”, which covers licensing to third parties and use by governments themselves. The latter is procedurally simpler because it does not require a prior attempt to negotiate a commercial license with the patent owner.
← 22. For the minority of WTO Members yet to accept the amendment, an interim waiver will continue to apply.