After reviewing selected ocean-related scientific and technological advances, Chapter 2 shifts to four individual in-depth case studies that serve to illustrate how some innovations in the ocean domain may potentially both enable economic development and support ecosystem improvement and preservation. The case studies include floating offshore wind power, ballast water management, innovation in the marine aquaculture sector, and possible conversion of decommissioned oil and gas rigs and renewables into artificial reefs.
Rethinking Innovation for a Sustainable Ocean Economy
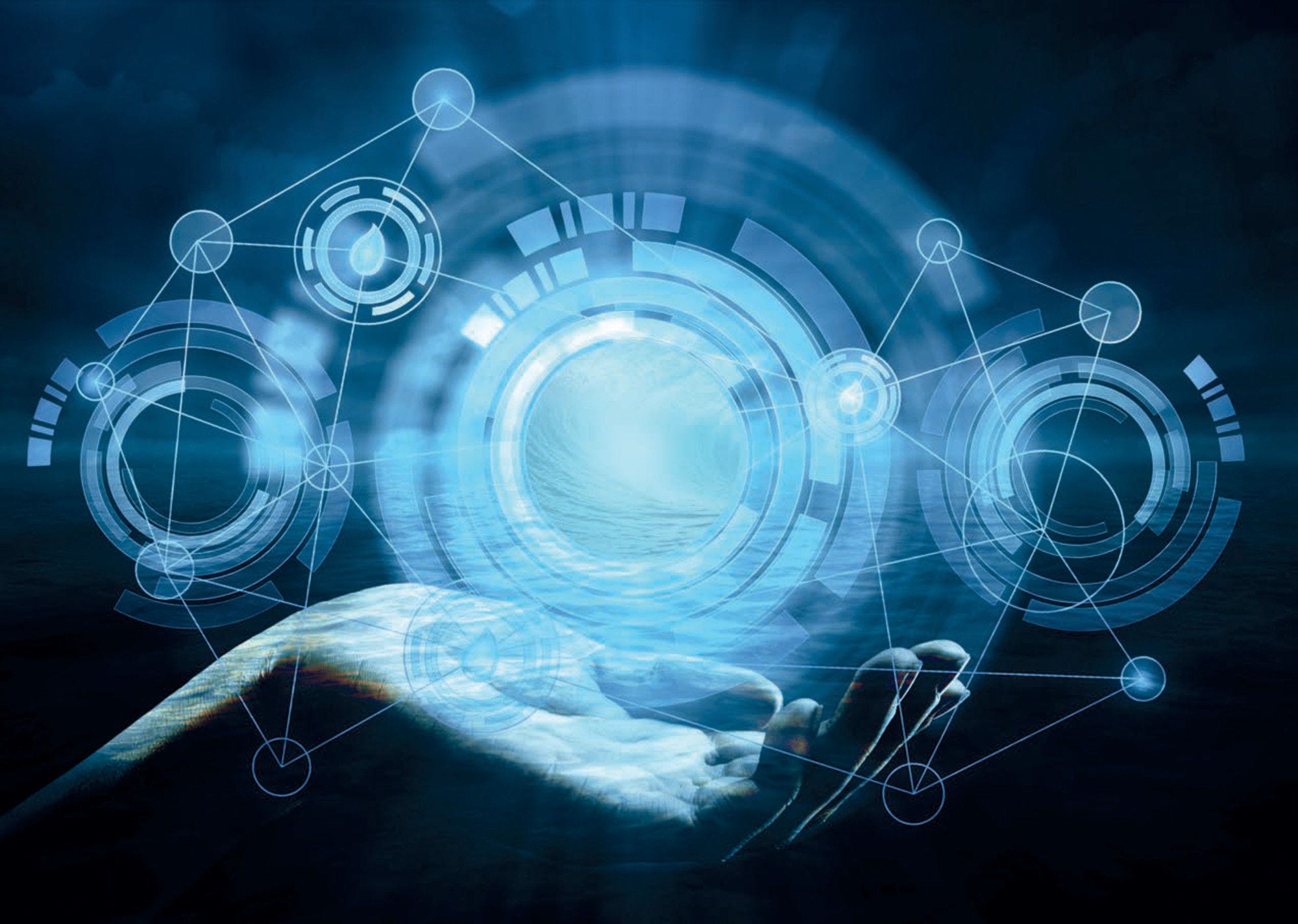
2. Science and technology enabling economic growth and ecosystems preservation
Abstract
2.1. Recent developments in science and technology
While the concept of sustainable or green growth is gaining ground in the ocean-user community, there is nonetheless continuing debate about the cost of trade-offs between economic development and environmental integrity involved in its implementation. This chapter offers insights into specific innovations and combinations of innovations, which can attain both objectives simultaneously: promote the economic development of ocean-based industries while fostering marine ecosystem sustainability.
With that context in mind, this OECD chapter has a threefold purpose:
Provide a brief update on ocean-related scientific and technological advances;
Contribute to the growing body of evidence indicating that the development of the ocean economy and sustainability of marine ecosystems can go hand in hand with one another;
And offer further insights into how science and technology innovation can contribute to improving that balance between economic development and environmental concerns.
After reviewing selected ocean-related scientific and technological advances, Chapter 2 shifts to four individual in-depth case studies that serve to illustrate how some innovations in the ocean domain may both enable economic development and support ecosystem preservation and improvement. The case studies include floating offshore wind power, conversion of decommissioned oil and gas rigs and renewables into artificial reefs, ballast water management, and innovation in the marine aquaculture sector. These case studies shed light on a trend in the ocean domain that is already emerging in many other areas of the economy, namely, the increasingly complex, rapidly changing, multifaceted nature of the challenges that science and technology are called on to address.
2.1.1. Ocean-sustainability challenges that science needs to address
Science is crucial to achieving global sustainability and adequate stewardship of our ocean, since it provides the wherewithal to deepen our understanding and monitor the ocean’s resources, its health, as well as predict changes in its status.
Recent years have seen the publication of numerous reports by national and international organisations setting out, from their standpoint, the main challenges and priorities that ocean science need to address. There are a good number of shared themes, for example: climate change and its impacts on the ocean (sea-level change, acidification, etc.), the deterioration of ocean and coastal ecosystems as a consequence of human activity, marine biodiversity loss, plastic pollution, declining fish stocks, ocean-related disasters, geohazards, and ocean governance, to name but a few.
However, there are also some divergences among the organisations in terms of national and regional priorities. For example, the impact on coastal communities and the issue of the Arctic are of key importance to the Canadian ocean scientific community’s report (Council of Canadian Academies, 2013[1]); the future of marine food webs figures prominently in the US National Research Council Decadal Survey of Ocean Sciences (National Research Council, 2015[2]); the European Marine Board identifies the need for a functional and dynamic definition of marine ecosystem health (European Marine Board, 2013[3]); and the uneven global distribution of benefits from the use of the ocean and its resources represent an important source of concern for the United Nations First Global Integrated Marine Assessment (United Nations, 2017[4]). A significant achievement of the UN Sustainable Development Goals for the ocean (SDG 14) was to capture for the first time and compress the essence of many diverse challenges facing the ocean science community (Box 2.1).
Box 2.1. Sustainable Development Goal 14 “Life below Water” with direct implications for science and technology
14.1 By 2025, prevent and significantly reduce marine pollution of all kinds, in particular from land-based activities, including marine debris and nutrient pollution.
14.2 By 2020, sustainably manage and protect marine and coastal ecosystems to avoid significant adverse impacts, including by strengthening their resilience, and take action for their restoration in order to achieve healthy and productive oceans.
14.3 Minimize and address the impacts of ocean acidification, including through enhanced scientific cooperation at all levels.
14.4 By 2020, effectively regulate harvesting and end overfishing, illegal, unreported and unregulated fishing and destructive fishing practices and implement science-based management plans, in order to restore fish stocks in the shortest time feasible, at least to levels that can produce maximum sustainable yield as determined by their biological characteristics.
14.5 By 2020, conserve at least 10% of coastal and marine areas, consistent with national and international law and based on the best available scientific information.
14.7 By 2030, increase the economic benefits to small island developing States and least developed countries from the sustainable use of marine resources, including through sustainable management of fisheries, aquaculture and tourism.
14.A Increase scientific knowledge, develop research capacity and transfer marine technology, taking into account the Intergovernmental Oceanographic Commission Criteria and Guidelines on the Transfer of Marine Technology, in order to improve ocean health and to enhance the contribution of marine biodiversity to the development of developing countries, in particular small island developing States and least developed countries.
Source: United Nations (2016[5]), Global Indicator Framework for the Sustainable Development Goals and Targets of the 2030 Agenda for Sustainable Development, United Nations Statistical Commission, 49th session, A/RES/71/313, New York, March.
One crucial element that all reports agree on is that the ocean is a very complex ecological and biogeochemical system that is under serious threat (e.g. pollution from human activity, overfishing in most parts of the world, destruction of marine ecosystems affecting the subsistence of local coastal populations) but which is still not well understood. How much pressure can the ocean take? How will an increasingly unhealthy ocean impact the planet’s biodiversity, the weather, the climate and our societies?
Two important interdisciplinary capabilities are still needed to get a better understanding of the ocean:
The capacity to study and integrate many diverse ecosystem dynamics and various biogeochemical cycles across different scales -- including temporal scales (in the order of days or centuries) and spatial scales (from a few kilometres to very large basins);
The capability to observe throughout the water column, all the way to the sea floor, the pressures and ecological-biogeochemical responses in the ocean interior (e.g. nutrients, oxygen, components of the plankton and indicators of their physiological status) to describe how very small processes could contribute to critically important and much wider variability.
This much needed multifaceted understanding of the ocean requires many scientific disciplines, from biology to physical sciences, to build up and analyse years of data from across broad expanses of the ocean, to collect new data, and deploy new technologies. International cooperation will be key, bearing in mind that organisational setups and ocean science capacities also vary greatly from country to country (IOC, 2017[6]). In addition to the different ocean science communities, other scientific disciplines are also joining in fundamental research that may ultimately benefit the ocean. For example, dedicated research and development into new sustainable petrochemical production routes (from production to use and disposal of products) may contribute to efforts to curb and stop the leakage of plastic pollution and other harmful chemical products into the ocean (IEA, 2018[7]). This primarily land-based pollution finds its way to the ocean via domestic and commercial wastewater (e.g. cleaning and sanitary products), agricultural run-off, and seepage from disposal sites. Much progress needs to be made to tackle the root of this chemical pollution, both in terms of the R&D required to find potential alternatives that would be less damaging to the environment, and in terms of changing current production and consumption practices (e.g. building on the circular economy concept) (OECD, 2018[8]).
Two milestones for the global ocean science community are forthcoming. For the first time, the Intergovernmental Panel on Climate Change (IPCC) will be producing a Special Report on the Ocean and Cryosphere in a Changing Climate in the second half of 2019 (IPCC, 2018[9]). Over 100 scientists from more than 30 countries are currently assessing the latest scientific knowledge about the physical science basis and impacts of climate change on ocean, coastal, polar and mountain ecosystems, and the human communities that depend on them. And 2021 will see the launch of the United Nations Decade of Ocean Science for Sustainable Development (2021-2030), with the objective that very diverse scientific communities and users of the ocean work together to develop further the knowledge base on the ocean (IOC, 2018[10]).
2.1.2. Selected trends in ocean science and technology
The OECD report on the ocean economy in 2030, written in 2015, already described many of the key advances under way in science and technology to help address the bulk of the challenges outlined above (OECD, 2016[11]).
In the course of the next couple of decades, a string of enabling technologies promises to stimulate improvements in efficiency, productivity and cost structures in many ocean activities, from scientific research and ecosystem analysis to shipping, energy, fisheries and tourism. These technologies include imaging and physical sensors, satellite technologies, advanced materials, information and communication technology (ICT), big data analytics, autonomous systems, biotechnology, nanotechnology and subsea engineering (Table 2.1). In addition to incremental innovations, there is the prospect of different technologies emerging and converging to bring about quite fundamental shifts in knowledge acquisition and marine industry practices.
Table 2.1. Selected incremental technologies and their use in the ocean economy
Incremental technology |
Expected use in ocean economy |
---|---|
Advance materials |
Capable of making structures stronger, lighter and more durable in offshore oil and gas, offshore wind, marine aquaculture, tidal energy etc. |
Nanotechnology |
Nano-scale materials that are self-diagnostic, self-healing and self-cleaning, used in coatings, energy storage and nano-electronics. |
Biotechnology (including genetics) |
Breeding of species, vaccine and food development in aquaculture. Development of new marine biochemical substances for pharmaceutical, cosmetic, food and feed use. Algal biofuels and new biomarine industries. |
Subsea engineering and technology |
Underwater grid technology, power transmission from deepwater, subsea power systems, pipeline safety, moorings and anchorings for floating structures, etc. |
Sensors and imaging |
Smart sensors, techniques and platforms that rely on miniaturisation and automation to create low-power, low-cost devices for measurement of the marine environment. |
Satellite technologies |
Improvements in optics, imagery, resolution of sensors, quality and quantity of satellite transmitted data, greater coverage by small, micro and nano-satellites, are expected to enable many activities. |
Computerisation and big data analytics |
Smart computing systems and machine learning algorithms set to make sense of vast amounts of data generated throughout the maritime economy. |
Autonomous systems |
Deployment of autonomous underwater vehicles (AUVs), remotely operated underwater vehicles (ROVs) and autonomous surface vehicles (ASVs) set to expand considerably. |
Source: OECD (2016[11]), The Ocean Economy in 2030, http://dx.doi.org/10.1787/9789264251724-en.
A cursory glance at the more recent literature suggests that, in the short period that has elapsed since the writing of the Ocean Economy in 2030 report, there has been an acceleration of research interest in the potential for applications of some of those technologies in gaining a better understanding of marine ecosystems, their workings, and the requirements for their better management (Box 2.2).
Box 2.2. Selected innovations enhancing knowledge of marine ecosystems, their management and their protection
Remote sensing using high-frequency radar and high-resolution satellites, with applications in:
Monitoring and modelling of oil spills from ships and offshore platforms, and of chemical contaminants (Singha and Ressel, 2016[12]; Li et al., 2016[13]; White et al., 2016[14]; Tornero and Hanke, 2016[15]; Strong and Elliott, 2017[16]; Spaulding, 2017[17]; Nevalainen, Helle and Vanhatalo, 2017[18]; Mussells, Dawson and Howell, 2017[19]; Azevedo et al., 2017[20]);
Measurement ocean surface currents using AIS (Guichoux, 2018[21]) and mapping global fishing activity (Kroodsma et al., 2018[22]);
Biochemical modelling of plankton biomass (Gomez et al., 2017[23])
Management of coastal zones and wetlands ( (Kim et al., 2017[24]; Wu, Zhou and Tian, 2017[25]).
Genetics, eDNA and other genetic toolkits applied in
Monitoring and assessment of (invasive) species in ecosystems (e.g. (Darling et al., 2017[26]));
Monitoring seabed mining disturbances (Boschen et al., 2016[27]);
Detecting bacteria in ballast water (Pereira et al., 2016[28]).
Acoustics, imagery and artificial intelligence advances in:
Monitoring (migratory) fish movements (Martignac et al., 2015[29]; Geoffroy et al., 2016[30]; Shafait et al., 2016[31]);
Monitoring marine habitats and ecosystem characteristics (Wall, Jech and McLean, 2016[32]; Cutter, Stierhoff and Demer, n.d.[33]; Trenkel, Handegard and Weber, 2016[34]); and recognition of marine species ( (Siddiqui et al., 2018[35]; Chardard, 2017[36]);
Autonomous systems. Progress in Unmanned Autonomous Vehicles (UAVs), gliders and Autonomous Underwater Vehicle (AUVs), including improved sensors, and high-resolution tools for 4D oceanic measurements:
Use of gliders in marine surveys (Colefax, Butcher and Kelaher, 2018[37]);
Use for research purposes in otherwise inaccessible and remote locations, including in the polar regions, and as key components of the Global Ocean Observing System (GOOS) (Forshaw, 2018[38]);
Monitoring and inspection in oil spill response (Dooly et al., 2016[39]; Gates, 2018[40]).
Enabling technologies appear set to contribute in important ways to the sustainable development of the ocean economy, not least by vastly improving data quality, data volumes, connectivity and communication from the depths of the sea, through the water column, and up to the surface for further transmission. While the examples are far from exhaustive, they do serve to illustrate the richness of current innovations in the ocean economy that are addressing either the one or the other objective. Two are particularly highlighted below:
Blockchain and big data analytics applications, for example, are starting to be deployed in port facilities and maritime supply chains. Shipping companies, logistics businesses, port operators and other maritime transport stakeholders are looking to more integrated services across the entire supply chain as a means of generating cost savings and greater efficiencies, as well as improvements in quality of service. The prospects for achieving those benefits by getting the various relevant operations (administration, logistics, shipping, terminal and port) to work together more smoothly have been boosted by the advent of digital platform technologies. For example, within the administrative segment of shipping operations, transport operators are currently exploring the potential for using distributed ledger technology (DLT), most notably blockchain. This technology does away with the need for an intermediary in transactions between stakeholders, while potentially offering a rapid and secure authentication method for freight transport. New players are emerging in the form of shipping tech start-ups with the capacity to leverage higher data volumes. They include digital freight forwarders, rate analytics services, collaboration or exchange platforms, tracking platforms and service fulfilment networks (International Transport Forum, 2018[41]).
The emergence of autonomous ships is also an important disruptive element for some industries, as are autonomous underwater vehicles (AUVs). AUVs and gliders with improved sensor platforms have progressed from niche status to an established part of operations in various marine sectors. In the oil and gas sector, however, the technology still has to mature to a stage at which oil and gas operators consider it a vital component of operations (Wilby, 2016[42]). This technology is deployed in monitoring and inspection for leakages in underwater carbon capture facilities, as well as in the inspection of deep-sea pipelines (Forshaw, 2018[38]). There are future opportunities for deployment in offshore decommissioning (Westwood Global Energy Group, 2018[43]) and possibilities for use in offshore wind (Westwood Global Energy Group, 2018[44]).
Other selected innovations primarily supporting the development of the sustainable commercial use of the seas and ocean are presented in Box 2.3.
Box 2.3. Selected innovations primarily supporting the development of the (sustainable) commercial use of the seas and ocean
Artificial intelligence applications in acoustics and imagery:
Development of machine learning to interpret subsurface images from seismic studies using computer vision technology and automate the analysis of technical documents with natural language processing technology (Zborowski, 2018[45])
Fisheries - Single beam and multi-beam echo sounder systems, real-time 3D visualisation software, sonars and catch monitoring systems (Kongsberg, 2017[46]), video imagery and recognition of fish species assisted by artificial intelligence (AI) (Siddiqui et al., 2018[35]);
AI in renewable ocean energy used for e.g. sea wave height prediction, sea-level variation prediction, wave hindcasting (Jha et al., 2017[47]).
Remote sensing using high-frequency radar and high-resolution satellites with applications in:
Decision making on suitability of aquaculture sites (Fernandez-Ordonez, Soria-Ruiz and Medina-Ramirez, 2015[48])
Offshore wind farms (Zecchetto, Zecchetto and Stefano, 2018[49]), (Kubryakov et al., 2018[50]).
Algal bloom forecasting for aquaculture insurance purposes (Miller, 2018[51]).
Modelling progress in applications in:
Development of methodologies and numerical modelling of wave and tidal energy and their environmental impacts (Side et al., 2017[52]; Venugopal, Nemalidinne and Vögler, 2017[53]; Heath et al., 2017[54]; Gallego et al., 2017[55]);
Wind resource assessments (Zheng et al., 2016[56]) (Kulkarni, Deo and Ghosh, 2018[57])
Impact of wind and waves on ocean facilities, e.g. siting of aquaculture facilities (Lader et al., 2017[58])
2.1.3. Introducing the four case studies
Beyond recent advances in science and technologies, the focus of this chapter is on innovations and combinations of innovations which embody the very the notion of green growth in the ocean by successfully fostering economic development and ocean sustainability simultaneously. To do this, it presents four in-depth innovation case studies: floating offshore wind; ballast water management; marine aquaculture; and rig- and renewables-to-reefs.
The four case studies are very different. They differ in scale and in the degree of maturity of the respective activity. Floating wind power is still in its infancy, with only one commercial-scale facility in operation in the world. Ballast water treatment technologies have so far been installed in only a small number of ships, but expansion could be rapid. Oil and gas rig conversion into artificial reefs is current in some parts of the world, but not in others, and no renewables-to-rigs programmes exist anywhere. Marine aquaculture, by contrast, is well established in many parts of the world, is undergoing rapid expansion, and is being transformed at great speed by a whole host of innovations. For this reason, the marine aquaculture case study is addressed in more detail and at greater length, in order to illustrate the sector-wide dimension of the innovation process.
Moreover, innovation in the four activities is driven by different forces and different challenges. Innovation in floating wind power is largely science and technology driven; rigs-to-reefs conversions are driven both by industry’s need to reduce decommissioning costs and conservationists’ desire to create or restore ocean ecosystems; innovation in ballast water treatment is propelled essentially by regulation; and innovation in the marine aquaculture sector is motivated by multiple factors: the challenge of feeding a growing world population, the incentive to develop business opportunities, and the need to reduce pressures on coastal environments. As different as the drivers may be, progress in all the cases is science-led or at least science-based.
Each case study endeavours to address a number of key issues: the background and global context; the economic and environmental issues at stake; the research and technological innovations in prospect; and the contribution that the innovations could make to enabling economic development within the sector (e.g. through cost-savings, new business generation, emergence of new industries) and environmental sustainability to go hand in hand.
2.2. Case Study 1: Innovation in floating offshore wind energy
2.2.1. The economic and environmental issues at stake
Until recently, floating offshore wind technology was largely confined to research and development, but the coming decades could see it emerge on a commercial scale. For that to happen, however, a wide range of technological, economic, regulatory and environmental challenges will need to be addressed.
The offshore wind industry has grown at an extraordinary rate over the last 20 years or so, from almost nothing to a total capacity of 18 GW in 2017. The way has been led mainly by Europe and China. Growth prospects are strong. Between 2017 and 2023, total global capacity is expected to almost triple to 52.1 GW (IEA, 2018[59]).
The potential economic benefits of such a rapid expansion are considerable. The OECD’s 2016 report on the Ocean Economy in 2030 suggests that, on a business-as-usual basis, the global gross value added generated by offshore wind power could increase eight-fold and employment more than twelve-fold between 2010 and 2030. As a consequence, its share of the global ocean economy could grow from less than 1% in 2010 to 8% in 2030 (OECD, 2016[11]).
Wind power also offers considerable benefits to the environment through its contribution to reducing global CO2 emissions. By way of illustration, estimates of carbon emissions from offshore wind generation conducted in 2015 place life-cycle emissions in the range of 7 to 23 g of CO2 equivalent per kilowatt (gCO2e/kWh). This compares with around 500 g for gas-fired conventional generation and about 1 000 g for coal-fired conventional generation (Thomson and Harrison, 2015[60]). A similar result was arrived at in a more recent study by Kadiyala et al. (2017[61]) which estimated the mean life-cycle emissions of the offshore wind installations surveyed at 12.9 gCO2e/kWh (with an environmental payback period of just 0.39 years).
Figure 2.1. Forecast of regional offshore cumulative capacity (GW) and wind generation (TWh) 2017-2023

Source: Adapted from IEA (2018[59]), Renewables 2018: Analysis and Forecasts to 2023, https://dx.doi.org/10.1787/re_mar-2018-en.
Moreover, the potential contribution to reducing CO2 output stands to benefit indirectly the world’s ocean ecosystems by helping to slow the increase in acidification, de-oxygenation and the rise of sea temperature and sea levels. Hence, further rapid expansion of offshore wind power suggests further gains for the environment in terms of CO2 reductions.
To date almost all offshore wind turbines are of the fixed-bottom type, installed in shallow water (up to around 50-60 meters) in proximity of the coast. Fixed-base wind turbines are arguably today successfully delivering low energy costs, improved energy security and low environmental impact, and much of the future growth in total offshore wind generation will continue to stem from this type of platform. However, over time, fewer near-coast shallow-water sites will become available due to growing competition for space from other ocean users, as well as to the lack of physically suitable locations. The latter applies in particular to countries like Japan and parts of North America and Latin America whose coastal zones are situated in deep water. Floating offshore wind platforms offer a way out of this dilemma. They could be installed in positions benefitting from more space, less potential interference with other ocean activities, and stronger more regular winds. In principle, the potential is very large indeed (Table 2.1).
Table 2.2. Potential for floating offshore wind power
Country/Region |
Share of offshore wind resource in +60 m depth |
Potential for floating wind capacity |
---|---|---|
Europe |
80% |
4 000 GW |
United States |
60% |
2 450 GW |
Japan |
80% |
500 GW |
Taiwan |
-- |
90 GW |
Source: Catapult and Carbon Trust (2017[62]) Floating Wind Joint Industry Project: Policy and Regulatory Appraisal, https://www.carbontrust.com/media/673978/wp1-flw-jip-policy-regulatory-appraisal_final_170120_clean.pdf.
Despite being able to draw quite heavily on the experience that the offshore oil and gas industry has with floating structures, seabed fixtures and related technologies, floating wind farms have taken some time to emerge as a commercial-scale installation. Indeed, to date, there is only one single large-scale offshore wind platform operating in the world: The 30 MW Hywind is situated off the Scottish coast and has been operating successfully – even surpassing expectations – since 2017 (Hill, 2018[63]). Nevertheless, at the time of writing, not one floating offshore technology has proved itself to the extent that it could be purchased off the shelf (Dvorak, 2018[64]).
However, many more projects are approaching fruition or are currently under development. The Scottish government has approved the 50 MW Kincardine Floating Offshore Wind Farm. The Council of Ministers in Portugal has given the go-ahead to the development of the 25 MW WindFloat Atlantic (WFA) project in Viana do Castelo, 20 km off the coast of Northern Portugal. France has approved a total of four pilot floating wind projects: a 24 MW pilot floating wind farm in the Gruissan area; a 24 MW floating wind farm planned by Eolfi and CGN for the Groix area; a 24 MW floating wind farm in the Faraman area; and another 24 MW project off Leucate. Work is also underway on the 2 MW Floatgen floating wind project in the Port of Saint-Nazaire. Japan installed the Fukushima Hamakaze floating wind turbine in 2016, and has initiated several further floating wind projects, such as the demonstration one off Kitakyushu. In the United States, the 12 MW New England Aqua Ventus I floating offshore wind demonstration project is under way in the Gulf of Maine. More developments are in the pipeline for projects off the coasts of Scotland, Wales and Ireland (offshoreWIND.biz, 2017[65]).
Consequently, expectations are running high that the market will develop rapidly in the coming decade, growing from almost nothing in 2017 to around 1 300 MW in 2030 and providing a total installed capacity of over 5 GW by 2030 (GWEC Global Wind Energy Council, 2017[66]). The basis for such optimism would appear to be, inter alia, recent progress in research, development and innovation.
2.2.2. Developments in research and technology
At the moment there are three designs of substructures under development: spar-buoy, spar-submersible, and tension leg platform (TLP); see IRENA (2016[67]), for more detail. A fourth foundation design – barge – is also well advanced, though less so than the others.
The high expectations of vigorous market expansion in the coming years are built on estimates of the potential for floating solutions and on the progress that has been made in bringing floating wind turbines up to an advanced level of technological readiness. According to Wind Europe (2017[68]), the spar design is already at technology readiness level (TLR) 9, and the others are expected to reach that score within the next five years: Expectations are further fuelled by advances that are being made in such areas as the siting of turbines, construction materials and methods, new designs, and monitoring and inspection, as the following examples illustrate.
Improved siting of turbines
Remote sensing is playing an increasingly important role as a decision support tool in choosing the most suitable locations for wind energy offshore. The key advantage of satellites over conventional surface observations is that satellite data can cover a wider spatial range, allowing for more comprehensive assessment of potential offshore wind energy resources. Zheng et al. (2016[56]) note, however, that there are a limited number of satellites and orbits, so that the data gathered may be inadequate in terms of time synchronisation and spatial resolution, as they may not be able to cover large surface areas at the same point in time during observation. Moreover, they cannot capture variations in the wind field at different altitudes. Satellite data can however be enhanced by applying numerical simulation methods to the energy resource evaluations. As a consequence of technological progress in modelling, recent years have seen more and more reanalysis data successfully deployed in this field. James et al. (2018[69]), for example, report on a successful modelling exercise using a three-year dataset of wind forecasts above ground level over offshore regions of the United States to support and improve energy resource assessments and wind forecasts for New England and other coastal regions of the United States.
Looking to the longer term, Zheng et al. (2016[56]) point to the need for improvements in a range of related fields, including: short-, medium- and long-term forecasting of wind energy; and early warning of natural disasters that pose a risk to wind farms. Over the even-longer term there is the issue of climate change and the uneven distribution around the globe of potential changes in the wind induced by global warming. Wind farms in some areas are likely to be impacted more severely than those in other regions. Here too more research is called for (Kulkarni, Deo and Ghosh, 2018[70]).
Improved construction materials and methods
As turbines have become ever larger over the years, so too have their rotor blades. They have increased in length quite considerably in recent years, from about 30 m in the 1990s to more than 100 m today. The blades themselves are composed mainly of fibreglass reinforced resins with add-ins of balsa wood and carbon fibre. The carbon fibre enhances the stability of larger turbines, thereby providing higher capacity. Thus, in future the share of carbon fibre is expected to rise, despite the fact that it is more expensive than other materials (McKenna, Ostman v.d. Leye and Fichtner, 2016[71]). Advances are also expected in meeting the challenge of the higher loads associated with larger rotors. Tests are under way on active load-control techniques such as trailing edge flaps and smart structures, intelligent pitch control systems, smart sensors to monitor blade load, and microtabs to modify the loads on the turbine structure. Other innovative concepts include smart blades that adapt to conditions and reduce or replace the requirement for active control (McKenna, Ostman v.d. Leye and Fichtner, 2016[71]).
The harsh conditions of the ocean climate place particular demands on the structure and workings of floating offshore wind energy platforms. Turbine blades are now manufactured from composite materials, in which further advances can be expected in the coming years. They need to remain in service for around 25 years, but are very vulnerable to rust and require regular inspection and treatment. Hence, preventive coatings for turbine blades are under development which aim to reduce substantially inspection and maintenance costs1 (Fraunhofer, 2016[72]).
Research is also under way to develop criteria to support decisions on the selection of materials for the foundations of floating wind farms. In the case of tension leg platforms, for example, various materials and methods are available but these tend to have different characteristics when it comes to lifecycle costs, CO2 emissions, etc. Kausche et al. (2018[73]) calculate that pre-fabricated steel or pre-stressed concrete have clear advantages over welded steel solutions, a research outcome that could apply equally to spar-buoy and semi-submersible type foundations, on which however to date no data have been published.
New designs
It is estimated that around 20 players are currently working to move new foundation designs from the concept stage to commercialisation (Renewable Energy Agency, 2016[67]). Numerous innovations are in the pipeline. The Spanish company Saitec, for example, is developing a cost-effective floating wind farm solution SATH (Swinging Around Twin Hull) fitted with two twin concrete hulls that are fastened to a single mooring point, allowing the platform to swing around. The economic advantages arise from the single mooring point system, which shortens installation times and facilitates returning to port for major work, as well as from the fact that the platform and turbine equipment are assembled on shore at the harbour, thereby minimising operations at sea (offshoreWIND.biz, 2017[65]).
Swedish specialist Hexicon is building platforms with floating foundations equipped with twin turbines for greater cost efficiency, and the platforms being designed by Swedish manufacturer TwinSwirl reduce manufacturing and maintenance costs by installing the parts requiring maintenance inside the easily accessible generator housing above water level (SeaTwirl, 2018[74]). On matters of grid connection, where one of the key problems is power transmission from a platform subject to significant movement, Japanese researchers have developed and demonstrated a dynamic cable system capable of stabilising power transmission (Taninoki Ryota et al., 2017[75]).
Looking to the longer-term horizon, designs are expected to progress from the single-rotor model deployed today to multi-turbine arrays on a single structure, generating as much as 20 MW of power from numerous 500 kW turbines and cutting both installation and maintenance costs. And as of 2040, the sector is expected to be making inroads into solving the weight-related problems posed by ever larger turbines on tower-structure designs. Vertical axis turbines, currently still in their infancy, offer considerable potential in this respect (Carbon Trust and Offshore Renewable Energy Catapult, 2017[76]).
It is noticeable however, that for many of these innovations, the timeframe is long and it is unclear how quickly they will find their way into commercial scale use.
Monitoring, inspection, maintenance and repair
Remote monitoring of conventional fixed-bottom wind energy facilities is already widespread, remote maintenance and repair operations on the other hand are still in their infancy. But as floating offshore wind becomes mainstream, platforms will increasingly be located in deeper waters and harsher conditions at ever greater distance from the coastline. As a consequence, installation, operation, maintenance, and repair will become more difficult, more dangerous and more expensive. Solutions will need to be sought in automated, remotely operated technologies. This should open up further opportunities for AUVs, ROVs, subsea robotics, and so on. Indeed, the United Kingdom’s Catapult (2017[76]) predicts that, a few decades from now, the deployment of drones and AI-driven monitoring systems for offshore wind farms – fixed and floating – is set to become commonplace, as is the use of remotely controlled and even autonomous underwater repairs and maintenance. Again, however, many of the above are unlikely to find their way into commercial scale application for many years yet.
2.2.3. Challenges facing floating wind technology
The high expectations with respect to the near and medium term development of floating wind technology need to be put in perspective, since there are many economic, technological, institutional and environmental challenges ahead.
While much can be learned from fixed-base offshore wind farms, floating wind technology is far from being a simple extension of that technology innovation system. The supply chain is different from that used for fixed-base turbines in shallow, near-shore waters; the technologies are different, as are the cost structures; the impact on the marine environment is different; and other ocean users may be affected in other ways (Bento and Fontes, 2017[77]). The difference in cost structure is particularly striking with respect to capital expenditure. While the foundations make up only 20% of total capex for conventional fixed-base offshore turbines (the main cost components are related to the turbines themselves), they account for around two-thirds of total capex in the case of tension-leg (TLP) floating wind platforms (Kausche et al., 2018[73]).
Costs figure among the most important potential barriers to rapid development of floating offshore wind farms. Higher average distance to shore correlates with higher wind speeds and higher capacity factors, but entails lengthier export connection cables to grid. Water depth usually increases with distance to shore, but is associated with higher installation and foundation costs, in particular mooring costs (Myhr et al., 2014[78]). Compensating cost savings may be expected from more integrated supply chains, economies of scale, upscaling of turbines, and major repairs conducted onshore, but most such savings will take time to come through. The longer term also holds further challenges. For example, grid integration costs are likely to increase as levels of penetration by variable power generation sources rise. The Levelised Cost of Energy (LCOE) for existing offshore wind farms in Europe in 2015 ranged from 7.3 €ct/kWh to 14.2 €ct/kWh, higher than the LCOE of onshore wind energy or conventional generation technologies (Höfling, 2016[79]). The LCOE of floating wind turbines, in turn, will very probably be higher than for both onshore and fixed-bottom offshore installations: according to the IEA, about 6% higher than the 2014 baseline in the IEA medium-term scenario (Wiser et al., 2016[80]). The results of the IEA’s survey of over 150 world experts in offshore wind power suggest that trend reductions in the LCOE of floating wind energy solutions could converge with those of fixed wind power platforms, but not until around 2030.
According to the findings of the IEA survey of experts, the most significant enabler for driving down the levelised cost of energy is – in addition to learning from experience – research and development. Significant technical innovations will be required to improve the competitivity of floating wind farms, but so will innovations in site identification, serial production, supply chain integration and management, and logistics during construction and operation.
2.2.4. Impacts on the environment and marine ecosystems
As noted earlier, the net contribution of floating wind energy to the reduction of CO2 emissions is significant compared to fossil-fuel based energy sources. There are nonetheless non-negligible drawbacks for the marine environment. These include the impact on (migrating) birdlife, the effects on fish and marine mammals, as well as those on the seabed and benthic habitats.
Most of the available research on ecosystem impacts draws on Northern European experience with fixed-base offshore turbines. Offshore structures can under certain circumstances enhance the marine environment due to increased biological productivity (of fish, molluscs and other forms of marine life). However, the construction phase would appear to be particularly invasive, with construction noise and disturbance of the seabed having effects on benthic habitats and many species of marine life, and once complete may in addition disrupt habitat connectivity both in and beyond the immediate area. Once in operation, the turbines can be a source of acoustic disturbance for a range of marine animals. There are also risks to bird life: potential flight displacement, risk of collision, and fragmentation of habitat by creating a barrier between birds’ nesting grounds and their feeding grounds. Some progress is being made in developing the technological and scientific tools to address some of these problems. In the case of risks to avian species, for example, techniques ranging from radar monitoring, Thermal Animal Detection Systems (TADS) and acoustic detection, to video surveillance and computational collision models are currently being deployed in the study of bird flight and behaviour in the proximity of offshore wind farms.
The view is emerging that, at least as concerns the construction/installation phase, floating wind turbines would be less intrusive during dredging and seabed preparation operations than fixed-bottom turbines (Carbon Trust and Offshore Renewable Energy Catapult, 2017[76]). Nonetheless, numerous issues remain. For example, for floating platforms, cable routes still need to be prepared and cables laid, activities that are associated with considerable disturbance of sediments. Also, the mooring systems for floating turbines need a large mooring spread. For a typical (catenary) mooring system, the spread diameter increases by 14 m with every 1 m increase in water depth. For a wind farm installed at a water depth of 150 m, for example, this would translate into a mooring system that required a 2 km diameter area of seabed for every turbine in the farm. Not only does that significantly raise the cost of mooring system materials, it also increases the lease area and expands the seabed environmental impact (Hurley, 2018[81]).
Also, the ornithological impact would depend greatly inter alia on how close to shore the floating facility is deployed. In relation to interaction of the floating foundation with fish and marine mammals, little research appears to be available, not least due to the fact that as of today few floating platforms exist.
The construction and operation of floating facilities should benefit from the experience of installing and running fixed-base wind turbines with ecosystem impact assessment. But even here, there are still large knowledge gaps. Lack of baseline data is a considerable impediment to the evaluation of impacts. Indeed, baseline research is needed on a whole range of natural phenomena including on marine species’ population structures and status, and their distribution and abundance over many annual cycles. And much remains to be done to improve the knowledge base on long-term ecosystem impacts as well as on the cumulative effect of multiple impacts. Similarly, information about the effects on the whole ecosystem (as opposed to those on single species or specific ecosystem components) is generally quite limited, although there are signs of progress; see for example Pezy, Raoux and Dauvin (2018[82]).
2.2.5. Concluding observations
With innovation evolving apace and levelised costs of energy projected to follow a downward trajectory similar to those of fixed-base wind platforms, a promising future is discernible for the floating wind energy sector. The growth potential is considerable, and from a broader ocean industry point of view, one would expect economic spill over benefits to flow to other maritime sectors such as ports, shipyards (construction and repair), marine equipment, and shipping. But given the many challenges this new sector faces, it seems unlikely that floating wind energy will be making a perceptible impact at commercial level in the near or medium term. Hence, also the indirect and direct benefits to the marine environment through displacement of fossil fuels will take time to have any effect. Science and technology are at the leading edge of developments to address the engineering and cost-reduction challenges, just as they will be required to play a key role in closing knowledge gaps and resolving many of the uncertainties still surrounding the environmental impacts of floating wind platforms.
2.3. Case Study 2: Marine invasive alien species and ballast water treatment
Marine invasive alien species have been spreading across the globe for centuries, often wreaking considerable damage on native ocean and coastal ecosystems. Ships have been the principal cause, and as globalisation in recent decades has gone hand in hand with rapid growth in maritime transport, so too has the dispersion of invasive alien species grown across the world’s oceans. Innovation in ballast water treatment may contribute to control this growing issue better.
2.3.1. The economic and environmental issues at stake
The marine invasive alien species’ problem is extraordinarily diverse and truly global in geographical scale, as the International Maritime Organisation’s (IMO) list of the world’s most troublesome invasive species indicates. In many instances, the impact on the environment has been devastating, depleting zooplankton stocks, competing with, displacing and sometimes wiping out local native marine life, disrupting food webs, obstructing coastal structures, and even posing a threat to public health. The environmental impacts are often associated with substantial economic costs, ranging from losses to fisheries and aquaculture, foregone revenues from tourism, deterioration of port facilities, and so on. The overall costs are thought to run into the USD tens of billions annually (King, 2016[83]).
Some examples of aquatic bio-invasions causing major impact are listed in the table below, but there are hundreds of other serious invasions, which have been recorded around the world (Table 2.3).
Table 2.3. Selected invasive aquatic species
Name |
Native to |
Introduced to |
Impact |
---|---|---|---|
Cholera Vibrio cholerae (various strains) |
Various strains with broad ranges |
South America, Gulf of Mexico and other areas |
Some cholera epidemics are reported to be associated with ballast water |
Cladoceran Water Flea Cercopagis pengoi |
Black and Caspian Seas |
Baltic Sea |
Reproduces to form very large populations that dominate the zooplankton community and clog fishing nets and trawls, with associated economic impacts. |
Chinese mitten crab Eiocheir sinensis |
Northern Asia |
Western Europe, Baltic Sea and west coast North America |
Undergoes mass migrations for reproductive purposes. Burrows into river banks and dykes causing erosion and siltation. Preys on native fish and invertebrate species, causing local extinctions during population outbreaks. Interferes with fishing activities. |
Toxic algae(red/brown/ green tides) various species |
Various species with broad ranges |
Several species have been transferred to new areas in ships’ ballast water |
May form harmful algae blooms. Depending on the species, can cause massive kills of marine life through oxygen depletion, release of toxins and/or mucus. Can foul beaches and impact tourism and recreation. Some species may contaminate filter-feeding shellfish and cause fisheries to be closed. Consumption of contaminated shellfish by humans may cause severe illness and death. |
Round goby Neogobius melanostomus |
Black, Azov and Caspian Seas |
Baltic Sea and North America |
Highly adaptable, invasive, increases in numbers and spreads quickly. Competes for food and habitat with native fishes, including commercially important species, preys on their eggs and young. Spawns multiple times per season, survives in poor water quality. |
North American comb jelly Mnemiopsis leidyi |
Eastern seaboard of the Americas |
Black, Azov and Caspian Seas |
Reproduces rapidly (self-fertilising hermaphrodite) under favourable conditions. Feeds excessively on zooplankton. Depletes zooplankton stocks; altering food web and ecosystem function. Contributed significantly to collapse of Black and Azov Sea fisheries in 1990s, with massive economic and social impact. Now threatens similar impact in Caspian Sea. |
North Pacific seastar Asterias amurensis |
Northern Pacific |
Southern Australia |
Reproduces in large numbers, reaching ‘plague’ proportions rapidly in invaded environments. Feeds on shellfish, including commercially valuable scallop, oyster and clam species. |
Zebra mussel Dreissena polymorpha |
Eastern Europe (Black Sea) |
Introduced to: western and northern Europe, including Ireland and Baltic Sea; eastern half of North America |
Fouls all available hard surfaces in mass numbers. Displaces native aquatic life. Alters habitat, ecosystem and food web. Causes severe fouling problems on infrastructure and vessels. Blocks water intake pipes, sluices and irrigation ditches. Economic costs to USA alone of around US$750 million to $1 billion between 1989 and 2000. |
Asian kelp Undaria pinnatifida |
Northern Asia |
Southern Australia, New Zealand, west Coast of the United States, Europe and Argentina |
Grows and spreads rapidly, both vegetatively and through dispersal of spores. Displaces native algae and marine life. Alters habitat, ecosystem and food web. May affect commercial shellfish stocks through space competition and alteration of habitat. |
European green crab Carcinus maenus |
European Atlantic coast |
Southern Australia, South Africa, the United States and Japan |
Highly adaptable and invasive. Resistant to predation due to hard shell. Competes with and displaces native crabs and becomes a dominant species in invaded areas. Consumes and depletes wide range of prey species. Alters inter-tidal rocky shore ecosystem. |
Source: IMO (2018[84]) Invasive Aquatic Species (IAS), http://www.imo.org/en/OurWork/Environment/BallastWaterManagement/Pages/AquaticInvasiveSpecies (AIS).aspx
The two main vectors by which marine invasive species are redistributed globally are biofouling (largely on ships’ hulls) and ballast water (Figure 2.2).
Figure 2.2. Biofouling and ballast water

Source: Fernandes et al. (2016[85]) Costs and benefits to European shipping of ballast-water and hull-fouling treatment: Impacts of native and non-indigenous species, http://dx.doi.org/10.1016/J.MARPOL.2015.11.015.
This case study focuses on the latter, namely the water that is used to maintain ships’ stability and integrity under different operational situations. As the size of merchant vessels increased and maritime traffic expanded over the last seventy years or so, the volume of untreated ballast water being transferred unintentionally from one location to another has grown enormously. It is thought that today tens of billions of tons of ballast water are carried between the world’s seas every year (Davidson et al., 2016[86]), spreading thousands of marine organisms – larvae, plankton, bacteria, microbes, small invertebrates, etc.- into the local marine ecosystem when discharged. This ballast-related redistribution of invasive species is widely recognised as a major environmental and economic problem, challenging, complex and currently still unresolved (Batista et al., 2017[87]). As a result, the need to treat ballast-water has become a priority initiative at global level, encompassing national efforts to strengthen regulation as well as multilateral efforts to design and implement an international convention.
2.3.2. Current state of regulation
International ballast water management regulation has been long in the making. Indeed, the International Maritime Organization (IMO) has been tackling it since the 1980s, when Member States of the organisation began alerting its Marine Environment Protection Committee (MEPC) to their concerns. The year 1991 saw the adoption of Guidelines to address the issue, followed by work to build the IMO Ballast Water Management Convention, adopted in 2004. The Convention was eventually ratified by the IMO in September 2016. Although scheduled to come into force on September 8, 2017, implementation has been delayed until 2019 (see Box 2.4). All ships to which the Convention applies should be equipped with a Ballast Water Management System (BWMS) no later than 8 September 2024.
Box 2.4. The Ballast Water Management Convention
The Ballast Water Management (BWM) Convention requires all ships in international trade to manage their ballast water and sediments, according to a ship-specific ballast water management plan. All ships must carry a ballast water record book and an International Ballast Water Management Certificate. Ship-based diffusion of organisms (including invasive species) may vary by vessel type. Moreover, there are significant knowledge gaps also with respect to the natural spreading of organisms.
“All ships engaged in international trade are required to manage their ballast water so as to avoid the introduction of alien species into coastal areas, including exchanging their ballast water or treating it using an approved ballast water management system.
Initially, there will be two different standards, corresponding to these two options.
The D-1 standard requires ships to exchange their ballast water in open seas, away from coastal waters. Ideally, this means at least 200 nautical miles from land and in water at least 200 metres deep. By doing this, fewer organisms will survive and so ships will be less likely to introduce potentially harmful species when they release the ballast water.
D-2 is a performance standard which specifies the maximum amount of viable organisms allowed to be discharged, including specified indicator microbes harmful to human health.
New ships must meet the D-2 standard from today while existing ships must initially meet the D-1 standard. An implementation timetable for the D-2 standard has been agreed, based on the date of the ship's International Oil Pollution Prevention Certificate (IOPPC) renewal survey, which must be undertaken at least every five years.
Eventually, all ships will have to conform to the D-2 standard. For most ships, this involves installing special equipment.”
Source: IMO (2017[88]) Global treaty to halt invasive aquatic species enters into force. http://www.imo.org/en/MediaCentre/PressBriefings/Pages/21-BWM-EIF.aspx
Full success of the Convention depends on a number of issues, the most important being the following:
It will only apply to ships from flag states having ratified the Convention, and to ships entering their jurisdictions. At present the treaty has been ratified by more than 60 countries representing more than 70% of world merchant shipping tonnage. Given that this includes the dominant shipping nations as well as a host of other countries, it would be practically impossible to sail internationally without complying with the Convention. This effectively puts a brake on the exchange of invasive species, as vessels from nations not complying with the Convention are restricted to their own territorial waters.
In view of the widespread lack of awareness about the environmental and economic consequences of ballast water, major efforts will be required to integrate and train ship crews and port staffs.
Further international negotiation and collaboration in the years ahead will be critical factors in consolidating, implementing and expanding the Convention.
2.3.3. Current state and future development of ballast water treatment technologies
The Ballast Water Management (BWM) Convention standard applies to water upon discharge. However, ship owners are fully aware of the biological growth in ballast tanks, and so many already have or will in future have procedures for ballast water treatment in place for both water uptake and discharge.
The design and operation of BWM systems face multiple challenges. They have to be usable for many different types of vessel, prove effective in destroying a wide range of organisms, and be able to operate efficiently and safely under all kinds of ballast water conditions such as varying ranges of salinity and temperatures. Consequently, research has given rise to hundreds of different BWM applications that use a variety of underlying technological principles. These currently range inter alia from ultra violet, oxidation and de-oxygenation, to electrolysis, ultrasound and heat, as well as chemical biocides. (Latarche, 2017[89]). However, among the many vessels already equipped with BWM systems that are approved for use by the IMO and United States Coast Guard (USCG) , the vast majority utilise two main technologies for disinfection purposes – electro-chlorination or ultraviolet irradiation – often in combination with filtration (Batista et al., 2017[87]).
Both technologies have been subject to criticism. For example, common and abundant seawater phytoplankton have frequently been found to be resistant to UV treatment; especially smaller organisms and microbes often survive; and so the question of population re-growth in the ballast tank persists (Davidson et al., 2017[90]; Batista et al., 2017[87]; Wollenhaupt, 2017[91]). Electro-chlorination, in turn, has been found to demonstrate lower disinfection efficiency in upper reaches of estuaries and freshwater surroundings because of their lower salinity (Batista et al., 2017[87]). Moreover, environmental concerns have been raised about problems related to pollution from some chemical treatments being applied to ballast water (Davidson et al., 2017[90]).
In the absence of more effective treatments today, what is in the innovation pipeline for tomorrow?
One solution may lie in the development of green, environmentally friendly treatments that can handle the double challenge of making significant inroads into the spread of invasive species at reasonable cost while at the same time reducing impacts on the marine environment. An example is pasteurisation technology that does without UV, filters and chemicals. The Danish Bawat BMS (2018[92]), for example, is aiming for USCG-type approval in the course of 2019.
Meanwhile, currently available technologies are undergoing continual improvement through evolutionary (rather than revolutionary) advances, and through innovations that aim to mitigate the shortcomings of existing techniques. To date, over 65 ballast treatment systems have been given type approval by the IMO. And in terms of mitigation (e.g. sampling), research is apparently generating numerous applications that allow testing for some organisms whose continuing presence post-treatment would involve severe penalties from the regulators. While such applications do not detect all organisms or bacteria identified by IMO or USCG regulations, they can provide indications of whether the treatment system is working effectively and allow the vessel operators to undertake further action. Increasingly, these are small, relatively inexpensive hand-held devices that detect the presence of viable algal organisms, microbial activity etc. in the ballast water. (Latarche, 2017[89]). In addition, advanced lab-on-chip detectors have been tested recently which can measure various characteristics of certain microorganisms in ballast water, for example number, size, shape and volume (Maw et al., 2018[93]), and detection of bacteria in ballast water by new-generation DNA techniques has also been successfully tested (Brinkmeyer, 2016[94]).
2.3.4. Estimating economic costs and benefits
In complying with the requirements of the Convention, there are clearly large potential gains for the environment. For the shipping industry however there are costs involved, in the form either of installing BWMS in the newly built vessels or in retrofitting existing vessels. The flipside of the coin is that the shipbuilding and maritime equipment sectors stand to benefit from the extra revenue this involves. But how large is the additional revenue likely to be?
Several attempts have been undertaken in recent years to estimate the size of the potential additional BWMS market. All of them necessarily entail building multiple scenarios because the many different types of ship of different size require different ballast water volumes and different equipment. In their work on the costs and benefits of biofouling and ballast water in the European market, Fernandes et al. (2016[95]) rely upon three categories of ballast water volume (< 1 500 m3, 1 500 to 5 000 m3, > 5 000 m3), five categories of vessels and four categories of tonnage. Splitting each of the three categories of ballast water volume into two categories of vessels accounts for 93% of the world fleet that uses ballast water. Creating categories in this way reduces the complexity of assessing the potential costs of measures across a range of vessels. For example, all ships with ballast waters volume of less than 1 500 m3 are passenger and fishing vessels of less than 10 000 tonnes.
Estimates of the number of ships in the global merchant fleet likely to install/retrofit ballast water treatment systems vary considerably depending on the model used, assumptions made, and possibly the juncture in the shipping/shipbuilding cycle at which the estimates were made. (King D.M. et al., 2012[96]) ventured a rough estimate of 68 000 vessels installing on-board BWTS between the time of writing and 2020, assuming full compliance of the Convention. Linder (2017[97]) suggests between 30 000 and 40 000 vessels could be candidates for retrofitting. Clarksons Research (2017[98]) projects potential retrofit demand at around 25 400 ships, plus 1 000 to 2 000 ships per year in newbuild demand. And the OECD (2017[99]) indicated around 27 000 to 37 000 existing vessels might be expected to retrofit BWMS between September 2017 and September 2024.
Estimates also vary when it comes to the average cost of retrofitting BWMS. Linder (2017[97]) estimates capital expenditure at USD 80 000-1 500 000, installation cost at USD 100 000-1 000 000, running cost at USD 0.01-0.02 per ton of ballast water, and sampling at USD 75 000-125 000 per vessel. Recent work of the OECD Secretariat (Figure 2.3) assess the impact of the Convention on additional demolition volume and retrofitting activity on the basis of three scenarios (OECD, 2017[99]). These are a high level scenario with installation costs reaching USD 3 million per ship; a medium level scenario with costs of USD 1.5 million per ship; and a low level scenario of costs of USD 0.5 million per ship.
Figure 2.3. Potential demand for retrofitting ballast water management systems under low, medium and high installation cost scenarios (number of vessels)

Source: OECD (2017[100]) Analysis of Selected Measures Promoting the Construction and Operation of Greener Ships, http://www.oecd.org/sti/shipbuilding.
A highly simplified calculation, taking 25 400 existing vessels at an average retrofit cost of USD 2 million, would suggest a potential global BWMS market in the order of USD 50 billion. [The figure is in line with estimates of USD 45 billion by Linder (2017[97]), and at the low end of estimates of USD 50-74 billion by King et al. (2012[96]), albeit for the period 2011-2016). What is clear, however, is that the Convention could have a significant impact on the ship repairing industries, generating business opportunities which – under the proviso of further analysis – “may occupy around 20% to 50% of retrofitting capacity in the coming 7 years” (OECD, 2017[99]).
How likely is it that the huge additional potential of the global BWMS market will materialise in the coming years?
Economic theory at least suggests that uptake could well be slower than expected. As noted earlier, concerns have been raised by the shipping industry about the effectiveness of many ballast water treatment systems and their ability to meet consistently IMO and USGC discharge standards. Furthermore, King (2016[83]) indicates that IMO guidelines for certifying and testing are considered by many observers as vague and open to interpretation, and that USGC is thought to be tending towards lowering BWMS testing standards in order to facilitate market access for USCG-certified BWMS. King (2016[83]) goes on to apply two well-established theoretical approaches to analyse the situation in the budding BWMS market. The first, based on work by the economists Akerlof, Spence and Stiglitz, indicates that “quality uncertainty” may prevent markets from developing or at least may result in poorer quality goods or processes, especially in regulation-driven markets. In the BWMS market, lack of strict quality criteria from the outset is likely to see bad quality replace good quality, thereby undermining a potentially successful regulatory regime. The second theoretical approach, namely “game theory”, draws on work by the economists Harsanyi, Nash and Selten, to explore inter alia how regulated industries react to such quality uncertainty by deploying strategies aimed at avoiding or delaying compliance, or reducing the costs of compliance. This would suggest that further action by governments and regulators will be required if an effective and viable regulatory regime is to emerge.
However, a counter-argument can be made to the effect that lowering the USCG standard may in fact prove beneficial, to the extent that it could result in one single common international ballast water standard. After all, the fact that the USCG standard is stricter than the UN IMO standard is thought to have caused uncertainties in the maritime industry – both among developers of ballast water treatment technologies and among ship owners – in respect of which standard to apply and which technology to purchase.
Finally, it should be noted that it may prove difficult to meet the requirements of the Ballast Water Management Convention within the proposed time range given both the limited conversion capacity and the corresponding costs for ship owners.
2.3.5. Concluding observations
Successful implementation of the BWMC, underpinned both by further improvement of conventional treatment technologies and by innovation in greener technologies (such as pasteurisation), holds out the prospect of substantial benefits to marine life and to biodiversity more generally in ocean ecosystems. Economic benefits should arise on multiple fronts: cost savings in the maintenance of coastal and port facilities, enhancement of native fishery stocks, reduction of harmful impacts on marine aquaculture, increased tourist revenues, to name but a few. Even the shipping sector, that undoubtedly shoulders the lion’s share of the costs associated with installation and retrofitting of ballast water treatment systems, ultimately also stands to gain as the knock-on effects expected from compulsory BWT feed through to lower levels of bio-fouling and consequently to fuel savings and lower emissions; see for example Fernandes, (2016[85]). However, it may prove difficult to meet the requirements of the BWMC within the proposed time range given the limited conversion capacity and the corresponding costs for ship owners. There is room for public policy both to encourage innovation in ballast water treatment and to ensure that a viable, effective regulatory system be implemented and sustained.
2.4. Case Study 3: Innovation in marine aquaculture
Marine aquaculture is a striking example of a sector in which scientific and technological innovations are combining in ways that can contribute significantly to both economic and marine-ecosystem sustainability.
2.4.1. The economic and environmental issues at stake
The importance of aquaculture has been growing rapidly in recent decades as an expanding world population, rising incomes and changing food consumption patterns have all combined to push up global demand for fish food. Moreover, with growth in capture fisheries having plateaued, future growth in seafood production is expected to come largely from aquaculture, further underlining its key role in global food security.
Marine aquaculture production – finfish, crustaceans, molluscs and aquatic plants – currently stands at about 59 million tonnes, equivalent to just over one-half of global aquaculture production (FAO, 2018[101]). It produces some 6.6 million tonnes of finfish, almost 5 million tonnes of crustaceans, and almost 17 million tonnes of molluscs (FAO, 2018[101]). The vast bulk of farmed aquatic plants consist of macro-algae or seaweed. At 30 million tonnes, they make up more than half of total marine aquaculture by weight, but in terms of value – USD 11.7 billion – their share is quite small (FAO, 2018[101]). Global production of seaweed has been growing quickly, more than doubling over the period 2005‑16, but remains dominated by China and Indonesia, which account for over 85% of the total (FAO, 2018[101]).
Future growth of aquaculture production volumes appears to be set on a slowing trajectory compared to the last few decades. The latest OECD-FAO Agricultural Outlook (OECD/FAO, 2018[102]) for example sees growth slowing to 2.1% p.a. in the period 2018‑27 compared to the 5.1% of the previous decade. Important contributing factors are the increasingly scarce raw materials for fish feed, limited availability of appropriate sites at current levels of technology, concern about the environmental footprint of aquaculture, and increasingly crowded coastal waters raising the risk of disputes among other ocean users (shipping, fishing, oil and gas etc.). In addition to these, the licensing environment (Innes, Martini and Leroy, 2017[103]) and national policy have a significant role to play. The development of “investment ready” aquaculture zones for example in places such as Australia show how national policy can encourage production. Conversely, China’s 13th five-year plan (2016‑20) is expected to slow growth in domestic production. Objectives there have shifted away from the past emphasis on increasing production and moved towards a more sustainable and market-oriented sector, where the focus is on improving quality and optimising industry structure. This will affect global aquaculture production due to the importance of China’s output at world level (OECD/FAO, 2018[102]).
Higher rates of growth in the coming decades are conceivable, but this would necessitate marked progress at several levels. Improvements in policy, licensing approaches and marine spatial planning could make important contributions. In addition, however, steps would need to include a reduction of the environmental impact of fish farms in coastal regions (e.g. destruction and/or pollution of coastal and aquatic ecosystems, introduction of exotic species into ecosystems, transmission of disease and parasites to wild populations), improved disease management, significantly higher proportions of non-fish feed for carnivorous species, and more rapid advances in the engineering and technologies required to establish offshore aquaculture operations (OECD, 2016[11]). In other words, future fish farming methods will need to be technically more sophisticated and more intelligent, requiring a “shift from experience-driven to knowledge-driven approaches” (Føre et al., 2018[104]). That in turn highlights the need also for scientific and technological innovation on multiple fronts.
2.4.2. Innovations in the pipeline
Innovation in aquaculture has in the past been largely technology driven. With some notable, albeit relatively recent exceptions, it has operated much through technology transfer, i.e. through mono-disciplinary research producing new technologies that emerge from the research system, get diffused by intermediaries such as advisory services, and adopted by users at farm level. Technology transfer is still regarded as the predominant approach to innovation in aquaculture, and is indeed considered the dominant culture in developing countries in South and Southeast Asia and Africa (Joffre et al., 2017[105]). While it has undoubtedly given rise to continuous innovation and remarkable increases in productivity, its practices have been subject to increasing criticism for what is considered to be inadequate consideration of ecological and social sustainability, and for creating growing challenges for ensuing generations of innovation processes. Systemic approaches on the other hand (e.g. systems innovation, socio-ecological approaches) which take a contextual, more holistic perspective on innovation, have become somewhat more widespread in recent years in developed countries. These tend to be multi-dimensional approaches, integrating technical, biophysical, environmental, economic and institutional dimensions into account (Joffre et al., 2017[105]).
In light of the above-described challenges and changes facing aquaculture development in the years ahead, especially for larger-scale intensive fish farming in developed countries, systemic approaches to innovation are moving increasingly into focus. Growing complexity of operation will call for novel solutions. In salmon farming, for example, direct observation as a means to garner key information on the state of populations and optimise their growth and welfare may become unviable for facilities housing millions of individual fish. And if, as expected, farms begin to move offshore2 into more exposed locations, access for personnel will become more problematic and remote automated operation more necessary (Føre et al., 2018[104]).
What kind of innovations are in the pipeline, which would contribute to addressing many of the challenges outlined above? What follows is an overview of advances unfolding in the various phases of aquaculture planning, implementation and operation, namely: aquaculture site selection, breeding, feeding, waste control, health monitoring and treatment, and engineering solutions.
Aquaculture site selection and area-wide assessment
Earth observation, with its exceptional spatial coverage, is considered to have substantial potential to support aquaculture management across a wide range of objectives, including aquaculture site selection, mapping of fish farm locations and surrounding area, as well as environmental monitoring (e.g. water quality) and the identification and tracking of toxic algal blooms (Kim et al., 2017[24]). While earth observation has been in use for some years in aquaculture research, recent advances in sensing can be expected to strengthen its contribution to improved aquaculture management in the years to come. Recent years have seen improvements in remote sensing with very high spatial resolution on board satellites programmed to revisit at shorter intervals, thereby expanding the capacity for tracking and monitoring sites. More such satellites are scheduled.
High data costs can of course limit access to advanced remotely sensed data, especially for developing countries, but here too the situation is evolving. Ever more high-resolution data from optical and radar satellites are becoming downloadable free of charge. The United States Geological Survey’s decision in 2008 to provide free access to its Landsat data archives proved ground-breaking in this respect. And more is on the horizon with the free and open data policy that applies to the Copernicus programme of the European Space Agency from its high resolution Sentinel-1A/B radar satellites and the optical Sentinel-2A/B sensors (Ottinger, Clauss and Kuenzer, 2016[106]).
Identifying appropriate sites for marine aquaculture is key to successful operation. On a smaller spatial scale than earth observation, a number of other useful tools exist for the purpose of site selection. These include for example GIS-based mapping, and modelling. GIS-based mapping affords access to marine and coastal datasets providing information for fish farm operators on availability of potential sites, as well on key factors such as environmental interactions and the presence of other, possibly competing ocean users. Recent progress has made it possible to combine GIS-based mapping with advanced modelling that provides further essential information such as predictions on oceanography, currents, animal growth, productivity and ecological environmental effects. The result is considerable enhancement of the outcome of the site-selection process by simultaneously augmenting farm productivity and reducing adverse impacts on the marine environment (Bricker et al., 2016[107]; Lader et al., 2017[58]).
Algal blooms figure among the greatest existential hazards to aquaculture. Around 300 different types of algal bloom have been identified, of which about one-quarter are toxic. When toxic algal blooms occur in shallow waters, they may pose a direct threat and can lead to severe reductions or complete loss of entire harvests. As non-toxic phenomena, they may also pose an indirect threat insofar as they can cause oxygen depletion (Ottinger, Clauss and Kuenzer, 2016[106]). In recent years, harmful algal blooms (HABs) of numerous varieties have occurred in a geographically wide range of waters: in North America (from New York to the Gulf of Mexico and the Pacific coasts of Canada and northwest United States), Scandinavia, Scotland, the Canary and Madeira Islands, and the Spanish and French Mediterranean, to name but a few. Harmful algal blooms are a natural occurrence in many areas and do not necessarily result from anthropogenic activity, and vary considerably over time, space and toxicity. Moreover, with growing climate change pressures, it is considered conceivable that harmful algal blooms may increase in frequency and severity (Wells et al., 2015[108]).
The economic cost of algal blooms is considerable. For example, in 2016 a series of HAB events caused severe damage to aquaculture in Chile. Salmon farmers lost some 39 million fish, equivalent to a harvest weight of 100 000 metric tons and a value of some USD 800 million (Anderson and Rense, 2016[109]). Shetland lost about half a million fish to one single harmful bloom in 2013. And until recently, the annual losses alone to the mussel industry in France, Ireland, Portugal, Spain and the United Kingdom totalled over EUR 30 million (Copernicus, 2016[110]). Looking to the future, harmful algal bloom events seem set to increase (Wells et al., 2015[108]).3 That, combined with expanding marine aquaculture capacity around the world, suggests the possibility of mounting economic costs in years to come unless science and technology begin to make substantial progress in developing an effective forecasting toolbox for early action (e.g. moving fish cages or reducing stocking density, or early or delayed harvest of shellfish).
The way forward consists in combining multiple approaches – earth observation (EO), in situ remote sensing, geographic information systems, new analytical tools for toxins, and mathematical modelling including algorithms. Although few in number, such systems that combine observation, modelling, etc. to predict harmful algal blooms do exist. In the United States, for example, the National Oceanic and Atmospheric Administration (NOAA) operates a forecasting system (HAB-OFS) for Florida and Texas that is based on a mixture of satellite imagery, field observations and mathematical models. A limitation is that it only identifies mono-specific high biomass blooms that can be detected from space with the help of ocean colour algorithms (Davidson et al., 2016[86]).
An additional example of such an integrated approach is the “ASIMUTH” project (Applied Simulations and Integrated Modelling for the Understanding of Toxic and Harmful Algal Blooms) set up as a response to the demand for short-term forecasts of harmful algal blooms events along the western European seaboard using earth observation (EO) data (Davidson et al., 2016[86]). Remote sensing satellite data and monitoring images track chlorophyll and water temperature. The system downscales the products of the Copernicus Marine Environment Service (CMEMS) and brings them together with biological data and input from harmful algal bloom experts to distribute warning bulletins to aquaculture producers via the internet or mobile devices. This permits the operators of fish and shellfish farms to make contingency plans (e.g. earlier harvest) to reduce the impact of the algal bloom event.
The benefits are twofold. Economic benefits are derived from accurate forecasting, and from timely action by farm operators. (Savings for mussel farmers in five major European aquaculture producing countries are estimated at over USD 2.5 million per annum (Copernicus, 2016[110]). Public health benefits are derived in the form of lower contamination rates of produce and lower impacts on human health.
Breeding
Selective breeding in aquaculture is generally aimed at raising profit rates through increasing harvest weight, improving disease resistance or shortening the time to harvest. Over recent years, breeding programmes at company level have shown that they can be highly profitable. In the case of sea bass or sea bream, for example, revenues typically surpass investment costs after five years and gross margins continue to increase thereafter. Selective breeding programmes for Atlantic salmon in Europe are estimated to raise production by 0.9% per year and additional profits by a total of almost EUR 31 million per annum. For sea bass, sea bream and turbot combined, extra annual production through selective breeding is estimated at 1 700 tonnes, and extra annual profits at EUR 2.7 million. Profits are expected to double in the coming years as more businesses launch selective breeding for sea bass and sea bream.
However, profitability alone may not remain the guiding principle in future, as concerns about the environmental impacts of marine aquaculture are growing. For example, excess feed and nutrient excretion contribute significantly to local eutrophication. Using European sea-cage farmed sea bass as an illustration, Besson et al. (2017[111]) show that the expected increase in sea bass production of almost 14% between 2014 and 2017 is likely to raise the rate of eutrophication by almost the same percentage, if nothing is undertaken to mitigate this. In addition, according to Komen (2017[112]), climate change can be expected to lead to rising and more extreme water temperatures, which in turn would exacerbate the problem.
In general, profitability and the potential environmental risks and impacts of marine aquaculture will vary considerably across regions and are influenced by a whole host of factors ranging from the number and density of cages and the species being farmed, to the specific ecology and environmental conditions of the farm location.
But there is clearly a place for selective breeding in future efforts to achieve a greater balance between business development and environmental sustainability. Increasing fish’s feed conversion ratios could considerably reduce the impact of excess nutrients on the environment while lowering feed costs and raising profitability. The economic and environmental sustainability of aquaculture already features among fish breeders’ key objectives (Besson et al., 2017[111]). But tomorrow’s breeding programmes will also need to consider the challenge of strengthening an animal’s resilience to changes in climate and environment, furnishing yet more incentives to re-orient breeding programmes towards environmental values.
In parallel with selective breeding, breakthroughs are emerging in genomics which foreshadow important potential improvements in the years ahead in fish breeding and aquaculture more generally. Underlying those breakthroughs are rapid advances in next-generation sequencing technologies and bioinformatics analysis of large sequencing datasets, leading to whole genome sequencing in aquaculture. According to Yue and Wang (2017[113]), more than 24 species have had their genome sequenced in at least draft form. Both GS and marker-assisted sequencing promise to bring about rapid improvements in broodstock and shorter breeding cycles in fingerlings, thereby accelerating the introduction of better species at farm level compared to what can be achieved through conventional selective breeding. Moreover, the latter should also eventually benefit from improved integration of molecular and genomics tools at the research and industrial levels. Yue and Wang also foresee progress in epigenomics (epigenetic modifications are reversible modifications on a cell’s DNA that affect gene expression without altering the DNA sequence) which cast more light on the interactions between the biochemical characteristics of marine organisms and environmental factors, thus contributing to both the sustainability and profitability of aquaculture4.
Feed
Feed is an extremely important input in marine aquaculture. It accounts for between 50 and 80% of total aquaculture production costs (FAO, 2017[114]). Moreover, feed can have a high impact on the environment in the form of waste (excess food, nutrient excretions, etc).
Significant progress has been made in recent years towards reducing or replacing fishmeal in the diets of many aquaculture species without compromising health or performance. Fish processing by-products, for example, currently make up around 25-30% of marine ingredients (Shepherd, Monroig and Tocher, 2017[115]). Efficiency increases are helping recover increasing quantities of oil and fishmeal from fish waste, along with increased demand for fillets which produces more waste. This means that production of fishmeal and fish oil from fish residue is expected to continue to rise (at rates of 2.8% and 1.6% per year, respectively) over 2018-27, taking the share of total fish oil obtained from waste fish from 39% to over 41% and that of fish-waste based fishmeal from 29% to more than 33% over the same period (OECD/FAO, 2018[102]). The consequences on the composition and quality of fishmeal and oil of increasingly relying on fish waste to produce them is also a source of uncertainty, as it will generally result in more minerals and less protein.
New ingredients for fishmeal include micro-algae, insect-based feeds, and plant-based feeds. Micro-algae are considered an environmentally sustainable alternative as they can be cultivated on a large scale and are not typically products destined for human consumption ( (Perez-Velazquez et al., 2018[116]; Henry et al., 2015[117]). Plant-based feeds include yeast-fermented rapeseed meal, soya, cereal grains, and so on. Results have been encouraging with respect to the proportions of fish meal replaced by plant-based inputs (Dossou et al., 2018[118]; Torrecillas et al., 2017[119]; Davidson et al., 2016[86]).
Similarly, much research has gone into the replacement of fish oil, not least because global fish oil supplies will not be able to satisfy the growing future demand for fish oil in aquafeeds, especially for carnivorous fish, and new alternative lipid sources are required to secure the future expansion of the aquaculture sector (Davidson et al., 2016[86]). Some of the studies indicate the possibility of high to very high replacement rates through vegetable oils (e.g. soybean, linseed, rapeseed, olive oil, palm oil, and so on) and algal-based oils across a wide range of fish and crustaceans, e.g. European seabass, red seabream, Atlantic salmon, red drum, rainbow trout and shrimp. For reviews of recent studies, see for example (Yıldız et al., 2018[120]; Torrecillas et al., 2017[119]; Shepherd, Monroig and Tocher, 2017[115]).
The effects on the composition of fish nutrition that the increasing replacement of marine products can bring about is illustrated very clearly in the farming of Norwegian salmon where between 1990 and 2013 the proportion of total marine sourced inputs fell from almost 90% to less than 30% (Figure 2.4).
Figure 2.4. Nutrient sources in Norwegian salmon farming feed resources from 1990 to 2013

Source: Ytrestøyl, Aas and Åsgård (2015[121]), Utilisation of feed resources in production of Atlantic salmon (Salmo salar) in Norway, http://dx.doi.org/10.1016/J.AQUACULTURE.2015.06.023.
In environmental terms, a downside has been that the fishmeal-free diet has been found to generate greater quantities of fish waste, notably total phosphorous and total suspended solids, as well as affecting oxygen demand in the water, although the poorer water quality did not negatively impact fish performance (Shepherd, Monroig and Tocher, 2017[115]). However, this is a matter that can be addressed inter alia by waste treatment technology (see following subsection).
On the economic front, the growing demand for fishmeal and fish oil replacements is translating into growing demand for alternative aquafeeds and supplements. The industry estimates the value of the global aquafeed market in 2017 at well over USD 100 billion and projects that figure to reach over USD 172 billion by 2022. That is equivalent to a compound average growth rate of around 10% (Research and Markets, 2017[122]).
Waste treatment
Marine aquaculture can affect the immediate ocean environment in different ways. As noted above, some types of farms (especially finfish) contribute to poor water quality through nutrition pollution and in some cases chemical pollution. The magnitude of the impacts depends on such factors as the species farmed, animal density in the cages, feeding method and location. Other types of aquaculture, notably algal and bivalves, have the potential to improve water quality: algae by taking up nitrogen, phosphorous and carbon, and bivalves by reducing phytoplankton and therefore eutrophication.
One systemic way to address the pollution problem associated with finfish farming is to introduce integrated multitrophic aquaculture (IMTA), which pairs different trophic levels of aquaculture in the same location with the aim of replicating natural ecological nutrient cycling. The fed fish produce excess organic matter which in turn feeds the bivalves and algae.
IMTA is widely acknowledged to hold considerable promise for significantly improving the sustainability of aquaculture, since it can potentially help achieve multiple objectives, including improved ecological efficiency, environmental acceptability, and profitability. However, while well established in many parts of Asia, IMTA is a much less familiar system to producers in Western countries where it faces numerous obstacles. In Europe, for example, challenges include lack of seed for promising local species, legislation issues, general lack of R&D knowledge, difficulties in handling the complexity of IMTA, and public acceptance (Kleitou, Kletou and David, 2018[123]).
There are however other innovations coming on stream which should be able to address many of the pollution issues facing marine aquaculture. These range from sensor platforms to detect uneaten food pellets falling to the cage bottom and algorithms to decide when the fish have had enough to feed, through procedures for improving food digestibility, to automated feeding techniques. In the latter case, technologies are available to observe behavioural variables in the fish; these include sonars, computer vision techniques, acoustic telemetry, spatial mathematical modelling combined with remote sensing, and artificial intelligence (Føre et al., 2018[104]).
Disease control and treatment
Of the many possible causes of aquaculture production losses suffered by aquaculture operators globally, disease is a major factor, accounting for around 40% of lost aquaculture production (Bastos Gomes et al., 2017[124]). Numerous outbreaks of disease in recent decades have proved particularly costly.5 Various innovations are in the pipeline to help address these problems.
a) Breeding for greater disease resistance
The worldwide increase in aquaculture production has given rise to many challenges including greater threats from diseases in both the freshwater and marine environments. Viral diseases such as infectious pancreatic necrosis, pancreas disease, cardiac myopathy syndrome, and heart and skeletal muscle inflammation have in the past wrought devastation on many a salmon farm. Sea lice for their part are considered the principal threat to sustainable salmon production around the world, having already inflicted significant economic and environmental harm. Selective breeding can reduce susceptibility to these pathogens, but conventional approaches require several generations to achieve results. In the case of Atlantic salmon, it is claimed that molecular genetics tools (e.g. MAS, genomic selection) will usher in major improvements in the accuracy and efficacy of procedures aimed at selecting for higher levels of resistance to pathogens and parasites (Norris, 2017[125]).
Eventually, with declines in sequencing costs and genotyping on the one hand and growing threats from climate change and pathogens on the other, it is expected that aquaculture science will move from genomic selection to genomic grading. In other words, it should become possible to tailor the genotype of a smolt to a specific location or even farm so as to optimise its survival (Norris, 2017[125]).
b) Prevention
Notable progress has been made in recent decades in controlling bacterial diseases – and therefore losses – in aquaculture through vaccination early on in the freshwater production stage. However, this does not apply to all regions and all species. Commercial development of vaccines for warm water fish and shrimp, for example, is still quite limited and in need of further development (Dadar et al., 2017[126]). Moreover, much less success has been achieved in tackling viruses and parasites (Norris, 2017[125]). Indeed, for some experts, most efforts in developing vaccines for aquatic animals are still very much in their infancy, and many hurdles will need to be overcome on the road towards multi-component, cost-effective vaccination programmes (Dadar et al., 2017[126]).
Success in prevention treatments will be vital to the sustainable growth of aquaculture at the rates required to meet rising demand for fish from an expanding world population. That focus on greater economic and environmental sustainability is one of the key drivers behind the aquaculture health sector, a business estimated to be already worth USD half a billion (Business Wire, 2016[127]).
c) Monitoring and detection:
Salmon farming is particularly susceptible to deadly outbreaks of the bacterial pathogen Piscirickettsia salmonis as well as the sea louse Caligus rogercresseyi. However, detecting them is in most cases both time-consuming and expensive. According to Føre et al. (2018[104]), this makes such an operation an excellent candidate for automation, deploying mainly optical tools. Spectral analysis can be used to distinguish sea lice from salmon skin, or hyperspectral analyses to detect alterations in the colour and texture of skin provoked by sea louse infestation. Estimations of sea lice numbers could then be constantly evaluated through an AI application that would compare the louse count to the legally set limits, and alert the farm operators when that limit is approached so that action can be taken. On this, see also Lopez Cortes et al. (2017[128]) and Gomes et al. (2017[124]) on the potential of eDNA approaches.
d) Treatment
Treatment (and in some cases prevention) of numerous pathogens has conventionally been through the application of chemotherapeutic approaches, finding widespread use in many aquaculture producing countries around the world, from Europe to Asia and Latin America. The obvious drawback of such techniques is that some chemicals may have negative effects both on the environment and on human health. Also widely used until some years ago were antibiotics. They too have been shown to have potentially serious drawbacks, notably by producing antibiotic resistance in the target pathogens, as well as raising concerns about the effects of prolonged use of antibiotics on human health in the form of antibacterial resistance. As a result, the administration of antibiotics in aquaculture is now strictly regulated in many countries, which has led to much sparser use of antibiotics (a notable exception being Chile in 2015). In turn, strategies for improved monitoring and prevention have also contributed to lower the necessity to use antibiotics. A frequently cited case is that of Norway where the use of antibiotics has fallen steeply to almost zero since the 1990s (Grave and Oslo, 2016[129]).
Attention has increasingly focussed on other means to treat outbreaks of disease. Among these is the growing interest in natural products. Studies are under way, for example, on the use of medicinal plants, marine algae, herbs and so on for disease management in fishes and prawns (Thanigaivel et al., 2016[130]). There is also further movement on the biotechnology front in biocontrol strategy research Defoirdt et al. (2011[131]) identify some promising novel biocontrol strategies that target the pathogens, e.g. bacteriophages that kill specific bacteria, bacterial growth inhibitors, and bacterial virulence inhibitors. At the time of that publication, the alternative biocontrol strategies discussed were still in the research phase and had not yet been tested in commercial aquaculture facilities.
A form of natural treatment of sea lice infestations which has gained considerably in popularity in salmon and rainbow trout farming is the use of cleaner fish – wrasse and lumpfish in Northern and North Western Europe, and potentially the robalo in Chile. Their use in Europe has increased enormously. Alone in Norway the numbers of cleaner fish used rose from tiny amounts in the 1990s to 1.8 million in 2000, to over 37 million in 2016. (OECD, 2017[99]; Norwegian Directorate of Fisheries, 2018[132])). The dynamics of cleaner fish use in salmonid aquaculture are a useful illustration of innovations that embody both economic development and environmental sustainability.6
Offshore aquaculture engineering and operations
A promising route for future expansion in the production of marine food is open-ocean aquaculture. It appears to offer numerous advantages compared to coastal seafood farming. These include fewer spatial constraints, less environmental impact, lower risk of conflicts with other ocean users, and fewer problems with disease. However, very few large-scale open-ocean farms are currently in operation, not least because they face a host of challenges: designing structures that can withstand the harsh conditions of the open ocean; access to the facility for monitoring, harvesting and maintenance purposes; communications; and safety of personnel, to name but a few.
Even assuming these challenges can be successfully addressed, just how big is the theoretical potential capacity for offshore aquaculture?
A recent study on the subject (Gentry et al., 2017[133]) suggests that the total potential area suitable for ocean aquaculture is immense. It estimates the area at over 11 million square kms for finfish and over 1.5 million kms for bivalves: sufficient to grow 15 billion tonnes of finfish a year, or 100 times the current global levels of seafood consumption. The process involves whittling down the total ocean surface available in continental regions at depths of up to 200 m and then mapping out and deducting a series of major constraints on the area suitable for growth: Marine Protected Areas, shipping traffic, oil rigs, areas of low chlorophyll concentrations (for bivalve cultures), and areas of low dissolved oxygen. Conservative thresholds were chosen for each of these variables, resulting in the elimination of some areas that may in fact be able to support marine aquaculture. Result: the area was reduced from 26 748 980 km2 to 11 402 629 km2 for fish and 1 501 709 km2 for bivalves. The final suitable global surface included both tropical and temperate countries, with many of the areas found to be located in warm, tropical regions. The total potential production is considerable: if all areas designated as suitable in this analysis were developed, the authors estimated that approximately 15 billion tonnes of finfish could be grown every year—over 100 times the current global seafood consumption.
Further economic, environmental and social constraints could come into play. These might include, for example, environmentally sensitive and/or high-biodiversity areas such as coral reefs, economic considerations like the distance to ports or access to markets, military zones, shoreside infrastructure, and intellectual or business capital. The social interactions with wild fisheries, jobs, prices and cultural heritage should also be taken into consideration. Other uses of these areas, such as by the military or for energy production, may also limit the available space. The result could be a further shrinking of the estimated area, but given the sheer scale of space available overall, there would still be ample room for adjusting future aquaculture sites to those additional constraints (Gentry et al., 2017[133]). A similar study by Oyinlola et al. (2018[134]), calculates a much larger area, but using a different methodological approach and fewer constraints.
Clearly, before such immense potential can be more fully exploited and scaled up in economically and environmentally sustainable ways, much more research will be required and many engineering solutions designed and field tested. Already many new models have been proposed7.
New designs are also likely to be much larger, containing millions of fish as opposed to thousands at present. Indeed, at the time of writing, some of them are close to completion and even operation. Foremost among these perhaps is Salmar’s Ocean Farm 1, installed off the Norwegian coast in 2017 and preparing for full operation later in 2018.8 An alternative concept is being developed by the United States company, InnovaSea, namely a set of submerged pens configured in a grid system and anchored to the ocean floor.9
In light of the size of the potential ocean surface available for marine seafood farming, the development of open ocean aquaculture on such a scale as sketched out here holds out the promise of huge business opportunities over the longer term which could at the same time make a major contribution to global fish farming sustainability. Those business opportunities are expected to emerge not only in the form of the revenues from efficient and sustainable growth of aquaculture itself, but also from the many new, smart economic activities that will form an integral part of the sector. For finfish, and to a large extent also algae (Kim et al., 2017[24]), these range from the mass development and installation of autonomous systems and technologies for remote operations as well as to complex decision support systems, to the design and construction of novel service vessels for exposed operations as well as autonomous and remotely operated undersea vehicles for site assessment, maintenance and repair.
However, before that era dawns, much scientific, technological and economic research across very many different disciplines will be required to pave the way. This applies no less to the environmental impact of open ocean aquaculture and the carrying capacity of ocean and seas. Since only few offshore farming projects are actually in operation around the world, data on ecosystem impact is very limited. That makes it particularly challenging to set a baseline of ecologically meaningful reference points such as minimum distance, depth, current velocity, etc. Without that data from impact analysis, the risks involved will first have to be explored using more theoretical model-based methods, perhaps along lines akin to those commonly applied in capture fisheries research (Froehlich et al., 2017[135]).
2.4.3. Concluding observations
If a large part of the many innovations described in this case study were to come on stream in the years ahead – especially those achieving a reduction in the environmental footprint of seafood farming – then marine aquaculture production could expand at more rapid rates than those generally projected. Since growth in the value of aquaculture production has been outstripping volume growth for some years now, the economic returns on innovations that help loosen the aforementioned constraints on output expansion could be substantial. Indeed, it could mean that gross value added of the global marine aquaculture sector could grow at well over 5% per year, trebling the sector’s value between 2011 and 2030 to around USD 11 billion (OECD, 2016[11]). Moreover, recent research indicates that there could in addition be positive knock-on effects further down the value chain. Input-output analysis for Ireland by Grealis et al. (2017[136]) suggests that expansion of aquaculture output could generate revenue and employment gains for both the aquaculture and seafood processing sectors on a significant scale.
2.5. Case Study 4: From rigs and renewable energy infrastructure to reefs
2.5.1. The economic and environmental issues at stake
Decommissioning of offshore oil and gas structures is a rapidly expanding industry with strong growth prospects. There are currently over 8 000 offshore oil and gas platforms in use around the world (OFS Research/Westwood Global Energy Group, 2018[137]), the vast bulk of them in the Gulf of Mexico and Western Europe .Close to 1 400 platforms operate in South East Asia and some 1 800 in the Asia Pacific region (Jagerroos and R Krause, 2016[138]).
Although several thousand platforms have already been decommissioned over the last few decades, principally in the Gulf of Mexico, the decommissioning industry is considered to be still very much in its infancy. The oil and gas industry currently decommissions around 120 structures annually (International Energy Agency, 2018[139]), the bulk of them in North America. This is set to continue apace over the coming years. It is estimated that more than 600 structures will be decommissioned over the 2016‑20 period, and a further 2 000 more between 2021 and 2040 (Cimino, 2017[140]; IHS Markit, 2016[141]). These estimates are broadly in line with those recently published by the IEA (2018[139]), namely between 2 500 and 3 000 offshore projects by 2040. While to date the bulk of decommissioning has occurred in North America, the next 25 years will see a much wider geographical spread of such activities, including Central and South America, Europe, Africa, Middle East, Eurasia and the Asian Pacific.
Moreover, it is mainly steel platforms in shallow water that have been decommissioned so far. In the coming years, however, many more deep-water installations and subsea tie backs (connecting subsea equipment on the seabed to the floating vessel or platform via risers) will be coming to the end of their service life. Such installations are more complex to decommission, signifying a steep increase in the engineering, financial and environmental challenges involved. As time goes on, decommissioning of wind turbines will add to the list of structures requiring removal as they reach the end of their life expectancy of 20‑30 years (Smyth et al., 2015[142]).
Technically speaking, a range of options is available for decommissioning offshore rigs which range from complete removal from the site to partial removal to conversion into an artificial reef (Table 2.4).
Table 2.4. Options for decommissioning offshore rigs
Options |
Description |
---|---|
Disposing at land |
Bringing the installation onshore, cleaning it, breaking it upinto scrap for recycling in the steel industry, or disposal at licensed sites. |
Toppling on site |
Cleaning the installation, placing or toppling the cut section on the seabed. |
Placing in deep water |
Cleaning the installation, and then towing it and placing it in a deep water site. |
Leaving on site |
Making the installation safe and leaving in-situ. |
Artificial reef |
Cleaning the installation and using it to form an artificial reef to improve local marine life. |
Re-use in another location |
Cleaning the installation, carrying out non-destructive tests, removing and transporting to another site suitable for the platform’s characteristics, then installing it at the new site. |
Re-use for another scope |
Making the installation safe and transferring use/purpose and potential ownership. |
Source: Shaw, Seares and Newman (2018[143]) Decommissioning offshore infrastructure: a review of stakeholder views and science priorities, http://www.wamsi.org.au/decommissioning-offshore-infrastructure-review-stakeholder-views-and-science-priorities.
The status quo approach to decommissioning prescribed in many countries is complete removal of the platform. That generally entails a series of steps by the oil and gas company to safely remove the structures: shutting down production, securely plugging and abandoning the subsea wells below the mudline, cleaning and removing all production and pipeline risers, removing the platform from its foundation, disposing of the platform on land in a scrapyard or fabrication yard, and ensuring that no debris remain that could potentially obstruct other users of the site location. This makes decommissioning an expensive business. The lion’s share of the expenses is often consumed by the plug and abandonment of the well, usually requiring a cement plug fitted by dedicated rig. Also very costly are the topside and substructure removal, transport and onshore deconstruction. Some cost savings may of course be achieved through the sale of the scrapped steel, whereby subsea materials in particular can prove to have high scrap value. There is also a growing interest in the circular economy, potentially making other cases of re-use more attractive, which should be taken into account when considering decommissioning. Estimates put the total decommissioning expenditure in Western Europe to 2040 at around USD 102 billion (Westwood Global Energy Group, 2018[144]), USD 100 billion in the Asia-Pacific region (Slav, 2018[145]), and around USD 40 billion for Australia (Khan, 2018, whereby a substantial proportion of the cost is likely to be borne by the public through tax concessions (Osmundsen and Tveterås, 2003[146]).
The environmental dimension of decommissioning is highly controversial, with decommissioning practice varying considerably across the globe. In regions where decommissioning is performed within a regulatory framework, the regulations often dictate that platforms should not be left standing and have to be removed. In some of these regions there is also a general public opinion, that the industry has a duty to restore, to the greatest extent, the environment to its original state. In other regions, however, there is a policy to encourage platforms to be left in place where appropriate (e.g. United States); in others (e.g. OSPAR) derogations are possible but are the exception. In yet others (e.g. Indonesia) no regulatory framework exists.
To a large extent, such differences are due to diverging views on the environmental impacts of partial or complete removal of structures. Indeed, the decision on how to decommission often entails difficult trade-offs. Partial removal of platforms which leaves substructures in place can for example lead to chemical contamination of the natural ecosystem or, in the case of intertidal offshore structures, favour conditions for the spread of invasive species. Complete removal, on the other hand, may result in contamination spreading beyond the site, threats to endangered species, destruction of benthic habitats and disruption of food webs.
At global level, little change to regulatory regimes appears to be on the horizon, with some notable exceptions (see further below). However, at expert level opinion may be shifting. The authors of a recent worldwide survey of experts by the Ecological Society of America (Fowler et al., 2018[147]) suggest that their findings support a growing global concern about the risks of infrastructure removal to the environment and marine ecosystems. While participating experts identified negative impacts for both partial and complete removal options, many considered the mass removal of infrastructure to be a potential source of new large-scale disturbance, and called for decommissioning options to be evaluated against a wider range of environmental issues, including biodiversity enhancement and provision of reef habitat, and protection from trawling.
In the final analysis, however, every case of decommissioning is governed by its own geophysical, financial, regulatory, technical and environmental specificities.
2.5.2. Converting oil and gas platforms into artificial reefs
Much as a result of the growing awareness of environmental impacts, but also of the possibilities opening up for ecosystem restoration, interest has been growing in recent years in converting redundant oil and gas platforms into artificial reefs.
Artificial reefs as such have been employed for centuries. A more recent phenomenon however is the design and implementation of plans and programmes to permit and indeed encourage the conversion of offshore structures into artificial reefs, known as rig-to-reef projects. A few such projects have been initiated in for example Thailand, Malaysia and Brunei. But it is in the United States that they are most firmly established, especially in the Gulf of Mexico (Figure 2.5). Indeed, the United States hosts the largest rigs-to-reefs project in the world, namely the Louisiana Artificial Reef Program. Responsibility for decommissioning offshore platforms lies with the Bureau of Ocean Energy Management, Regulation and Enforcement (BOEM), in consultation with state authorities and several federal agencies including the Environmental Protection Agency, NOAA, the Army Corps of Engineers, Fisheries, and the Coast Guard.
Figure 2.5. How the Rigs-to-Reefs Programme works in the United States

Source: Grasso (2017[148]) Rigs-to-Reefs Program, presentation at the OECD Workshop on Innovation for a Sustainable Ocean Economy, October 10-11, 2017, Naples, Italy.
Not all platforms are suitable for conversion. Eligibility for transformation is subject to engineering standards, and numerous criteria play into the decision making process, including the size, structural integrity and location of the platform. In general, the preferred candidates for reefing are complex, stable and clean structures (BSEE, 2015[149]). By 2016, a total of 516 platforms had been converted into reefs, equivalent to around 11% of all platforms decommissioned in the Gulf of Mexico since 1987 (Grasso, 2017[148]).
United States practice offers insights into how savings and economic benefits come into play in the conversion process. For the oil and gas company, the economic incentive consists in the difference between complete removal of the platform and only partial removal for the purposes of reef conversion. The monetary benefit for the environment is that around half of that saving by the oil and gas company goes to the government’s artificial reef programme to support the creation of the reef.
Assessing the ecosystem benefits of rig-to-reef programmes
How successful so far are rig-to-reef programmes in terms of enhancing or restoring fish and mollusc populations and biodiversity more generally? As noted above, the most comprehensive platform conversion programme has been conducted in United States waters (Gulf of Mexico and Pacific), where it has generated considerable research into this question. For the Gulf of Mexico, it appears that the number of structures on a given reefing site may have considerable impacts on the density of some species (Ajemian et al., 2015[150]), in this particular case on the stocks of economically important red snapper. (Claisse et al., 2014[151]) focussed on waters off the coast of California to compare annual secondary production of fish communities on oil and gas structures to those on natural reefs as well as to secondary production estimates of fish communities from other marine ecosystems. (Secondary production is the formation of new animal biomass from growth for all individuals in a given area during some period of time). They found that “oil and gas platforms off the coast of California have the highest secondary fish production per unit area of seafloor of any marine habitat that has been studied, about an order of magnitude higher than fish communities from other marine ecosystems.”
However, Caisse et al (2014[151]) also raise the much-debated question of whether these structures produce a net addition to fish populations in the area, or whether they merely attract existing fish populations from the surrounding area. (This is important since such an accumulation of fish around hard structures may attract more intensive fishing, possibly leading to over-exploitation of stocks in the long term.) In the case of Californian waters, they concluded that “the platform was not drawing fish away from recruiting to other natural habitats, but providing a net increase in recruitment.” They noted however that this finding was unlikely to apply to all species and all platforms. For the Mediterranean’s largest artificial reef (Cresson, Ruitton and Harmelin-Vivien, 2014[152]), also found that non-natural reefs can contribute to local secondary production. Jagerroos and Krause (2016[138]) too confirm that new reef habitat indisputably increases the abundance of the local fish and invertebrate communities by functioning as a fish aggregation device, but also they raise the issue of “attraction” versus “stock enhancement”.
As indicated earlier, not many regions of the world have decommissioning regulations in place, as in the United States, which take a flexible if not favourable approach to rig-to-reef transformation. The North Sea as part of OSPAR is a case in point, where there has never been a rigs-to-reef programme. However, in recent years a series of scientific studies have indicated that rig conversion to reefs may well be an appropriate strategy for fish conservation in the North Sea, as it can have positive effects on deep-sea benthic communities, function as safe harbours for some fish stocks, and host cold-water coral colonies. The upcoming stream of decommissioning projects provides an opportunity to initiate the creation of large-scale reef systems to benefit ocean life (Jørgensen, 2012[153]). As of 2017 no change to OSPAR guidelines is in sight, but pressure is growing from industry, the scientific community and conservationist groups in support of reusing rigs for reefs in the North Sea; see for example Porritt (2017[154]).
It may however prove difficult to bring about significant widespread change. The Norwegian shelf, for example, currently counts 12 concrete facilities, 23 steel floating facilities and 59 steel facilities resting on the seabed, in addition to which there are nearly 400 subsea installations. The authorities have at present approved a total of about 20 decommissioning plans, six of them in the last two years. Over the next five years, up to one-quarter of the fields currently on stream could be closed. But the rules and regulations governing the Norwegian Continental shelf dictate that facilities must be removed in their entirety; only in extremely limited cases can they be abandoned on the field once they have reached the end of their useful life (Norsk Petroleum, 2018[155]). Some margin of manoeuvre may be found in the case of deep-sea oil and gas fields deploying floating installations. The anchoring structures and cable connections could be used for offshore wind farms, or indeed for future deep-sea fish farming.
What emerges from the many studies conducted so far then is that, while the United States experience in particular seems to have been broadly very positive, it remains unclear whether that experience is easily transferable to other regions, locations, and other environments (Techera and Chandler, 2015[156]). As Jagerroos and Krause (2016[138]) note, some offshore platforms that have been in operation for many years have not fostered the diversity of benthic or fish communities comparable to that which can be found on some natural reefs. Indeed, every site reveals different characteristics in terms of marine life, substrate, currents, proximity to natural reefs, and so on (Lyons et al., 2013[157]).
A growing role for science in assessing suitability for rig conversion
The widespread scientific view today is that rig conversion programmes can only be viable after a thorough prior assessment of the many factors and criteria determining their success. These include the composition of marine life on the site but also further afield, the environmental risks, biodiversity conservation issues, questions of location, priority setting, potential benefits and trade-offs, and matters of stakeholder involvement.
This applies no less to the North Sea for which there is a serious lack of data to understand how man-made structures interact and influence its ecosystems (UK Department for Business, 2018[158]) and for which major international research efforts are required. The European-financed INSITE project is one such undertaking. Its aim is to help establish the magnitude of the effects of man-made structures on the North Sea ecosystem, considered on different time and space scales, and to establish to what extent, if any, the man-made structures in the North Sea represent a large inter-connected hard substrate ecosystem (INSITE International Scientific Advisory Board ISAB, 2018[159]). Results so far indicate that oil and gas structures do indeed create a network of hard substrate ecosystems for certain species, and that they may act as bridges (via larval dispersion) between otherwise distinct networks, be they in the deep sea, in fjords or in marine protected coral areas (Henry et al., 2017[160]). In practical terms, the work to date has shown that the network analysis modelling tools used by the project could be useful in supporting decisions on decommissioning. It also demonstrated the value of DNA barcoding and population fingerprinting in supporting connectivity modelling for specific species.
Other regions of the world too are becoming increasingly aware of the potential benefits of rig conversion on the one hand, but also of the scientific research effort required on the other. In Australia, for example, the National Offshore Petroleum Safety and Environmental Management Authority has been exploring such decommissioning options, and considerable scientific analysis is under way into rig-to-reef solutions, not least in light of the water depths at which many oil and gas facilities operate, and also in view of the uncertainties surrounding monitoring obligations. Under the new decommissioning guidelines published in January 2018, “options other than complete removal may be considered, however the titleholder must demonstrate that the alternative decommissioning approach delivers equal or better environmental, safety and well integrity outcomes compared to complete removal” (Department of Industry of the Australian Government, 2018[161]).
Also, in January 2018 the Western Australia Marine Science Institute published its findings from a wide ranging stakeholder consultation on decommissioning issues (Shaw, Seares and Newman, 2018[143]). Among the headline implications drawn from the project was confirmation that:
“there are knowledge gaps that need to be addressed through science before decision-makers and stakeholders are able to efficiently and effectively consider the full range of decommissioning options as a matter of normal practice
Confidence of short and long term environmental risk and/or acceptability of different decommissioning options is the overriding priority for stakeholders”.
In Southeast Asia around half of the 1 700 oil and gas facilities in operation are more than 20 years old and approaching retirement, and studies are under way in several countries on the feasibility of converting platforms into artificial reefs (Jagerroos and R Krause, 2016[138]).
2.5.3. From offshore wind platforms to reefs
There is also potential for transforming fixed-bottom offshore wind platforms into artificial reefs. As pointed out previously, the offshore wind industry has witnessed spectacular growth over the last couple of decades, from almost nothing to a total capacity of 14 GW in 2016. Growth in the medium-term future is also well on track, with total global capacity expected to triple to well over 40 GW by 2022. The longer-term prospects suggest a further tripling of capacity to around 120 GW by 2030, the vast majority of which will be fixed-bottom installations (GWEC Global Wind Energy Council, 2017[66]).
Offshore wind installations have an expected service life of around 25 years. Those that started operating in the early years of the 21st century are expected to be decommissioned in the course of the 2020s. However, it will be a number of years still before decommissioning will be required on a major scale. When, and how many, will depend on a variety of factors, including the advent of new technologies, the identification of more suitable sites, and the cost of equipment upgrades, all of which may render many existing installations uneconomical sooner rather than later (International Energy Agency, 2018[139]).
In light of those prospects, and reflecting the largely positive experience of North American conversion of oil and gas installations into artificial reefs, attention is now turning to the potential for converting also offshore wind platforms into artificial reefs. For legal, financial and environmental reasons, decommissioning requirements are usually an integral part of licensing and consent for all marine developments, and offshore wind is no exception – the options for offshore wind structures being either full removal, or partial removal leaving some elements in place. While the overall structure of offshore wind platforms is different from that of offshore oil and gas rigs, it is thought that the general principles of conversion into artificial reefs apply, including the potential benefits to ecosystem conservation and development, fisheries and recreational activities. A major difference does pertain, however, insofar as oil and gas rigs may be installed in deep water, thereby presenting fewer navigational risks than wind farms located in shallow waters. But it can be argued that such risks may be outweighed not only by the ecosystem benefits attached to partial removal, but also by the lower energy and labour costs as well as reduced safety risks compared to full removal (Smyth et al., 2015[142]).
Similar to rigs-to-reefs, there are issues around the net benefits of offshore wind platform conversion that would accrue to the ecosystem in terms of new marine life production. To date, very few wind farms have been decommissioned, and so evidence is limited. However, numerous studies indicate increased species abundance around offshore wind foundations, and they are typically associated with positive effects for the local ecosystem; see for example (Bergström et al., 2014[162]). Using improved data and advanced modelling techniques, some scientists have recently begun to link the projected expansion of offshore wind farms with future impacts on certain marine species. Slavik et al (2018[163]), for example, anticipate that on completion of all planned offshore wind installations in the southern North Sea, the overall abundance of the blue mussel will have increased by more than 40%, providing an additional food source (also for some crustaceans), and benefitting ecosystem functioning thanks to their filtering of phytoplankton. Examining offshore wind projects for the Bay of Seine (English Channel) ecosystem, Raoux et al. (2017[164])) projected the potential impacts of benthos and fish aggregation resulting from the installation of the concrete piles and the turbine scour protections. Their results suggest an increase in total ecosystem activity as well as in some fish species, marine mammals and seabirds as a reaction to the biomass aggregation around the infrastructures.
What is clear already at this early stage is that the long-term effects of offshore wind farms on local marine ecosystems as well as on ecosystems further afield are still unknown. If the environmental and economic potential of decommissioned offshore wind farms as artificial reefs is to be fully captured, much more scientific evidence will need to be gathered and evaluated.
2.5.4. Concluding observations
Experience to date, gathered primarily in the Gulf of Mexico and the Pacific since 1987, suggests that only a fraction (around 10%) of oil and gas rigs is suitable for conversion to artificial reefs. Nonetheless, in light of the thousands of rigs to be decommissioned in the coming decades around the world, the number of potential future conversions is significant. Yet, while the United States has accumulated some 30 years of experience with rig-to-reef conversions, most other areas of the globe have been slow to follow, not least because rig-to-reef conversion is not easily transferable from one region to another. Indeed, suitability of rig-to-reef conversion is highly site-specific. Moreover, in many cases, the concern has prevailed that leaving part of the rig infrastructure in place risks serious pollution of the marine environment and that complete removal of the infrastructure should be required; in many other cases, no regulatory framework even exists and needs to be developed. However, the decommissioning issue is increasingly controversial. Support among marine scientists and conservationists is growing in favour of the view that in some instances, complete removal of the platform may cause more harm to the marine ecosystem than leaving the lower structure in place, and that, unless serious pollution risks exist or maritime traffic safety is threatened, some consideration should be given to partial removal.
The economic effects of only partial removal tend to be significant and immediate. The oil and gas companies (and, in the longer term future, offshore wind operators) stand to make significant savings from the reduced decommissioning operation. Governments do however have the option – as they do in the United States, for example – of requiring the companies to pay a specified share of those savings into a fund for reef conversion. (It should be noted however that governments may still be open to criticism from conservationist quarters for allowing oil and gas companies to gain financially from such a reduction in clean-up effort.) There are also likely to be positive effects for the marine environment resulting from less disturbance of the seabed and the water column, which can translate into improved stocks of fish, molluscs and so on.
A potentially positive long-term economic contribution may also be associated with the decision to convert to artificial reefs. Healthy artificial-reef ecosystems can make for more productive commercial fisheries, attract tourism, diving, and recreational fishing, while the decommissioned structure itself may come to serve multiple alternative purposes in support, for example, of aquaculture facilities or marine renewable energy (OECD, 2016[11]). It should be noted though that benefits to the fisheries may depend upon the type of fisheries undertaken (Multiconsult, 2011[165]). Moreover, there are potential positive spill-overs for other sectors. For example, specialised engineering companies stand to profit from the business generated by the creation of the artificial reefs; see for example Smyth et al (2015[142]), on subsea eco-engineering, and Offshore Digital Magazine (2017[166]), on emerging rigs-to-reefs business partnerships. And even insurance and re-insurance companies look set to engage in the business of ecosystem resilience and restoration (Leber, 2018[167]).
In addition, the growing volume of scientific assessment, inspection and monitoring involved in the decision-making and implementation of rig and (eventually) wind turbine conversions promises to offer considerable opportunities for the owners and operators of AUVs, ROVs and other subsea vehicles. The sector, while still small, is currently expanding rapidly. The latest world AUV forecast (Westwood Global Energy Group, 2018[44]) for example, sees total global demand increasing by almost 40% between 2018 and 2022. Demand growth in the research sector is still modest, but is nonetheless expected to see its share of total demand reach 25% by 2022. Commercial demand should see the fastest growth rates, expanding by 74% over the next five years.
Rigs-to-reef programmes and renewables-to-reef projects are at very different stages of development. Yet both offer long-term potential for synergies between economic gain and ecosystem benefit. Neither will be able to unfold that potential to its full extent unless long-term strategies, appropriate incentives and effective regulatory frameworks are put in place by public authorities working closely with the private sector and the many other stakeholders concerned. Such strategic policy decisions and collective actions, however, need to be founded on the best possible scientific evidence with respect both to the environmental issues surrounding the debate of partial versus complete removal of infrastructures, and to the question of the successful creation and long-term viability of artificial reefs. As this case study has endeavoured to show, much work is still required from the scientific community to deliver that evidence.
References
[171] Abolofia, J., F. Asche and J. Wilen (2017), “The Cost of Lice: Quantifying the Impacts of Parasitic Sea Lice on Farmed Salmon”, Marine Resource Economics, Vol. 32/3, pp. 329-349, http://dx.doi.org/10.1086/691981.
[150] Ajemian, M. et al. (2015), “An Analysis of Artificial Reef Fish Community Structure along the Northwestern Gulf of Mexico Shelf: Potential Impacts of “Rigs-to-Reefs” Programs”, PLOS ONE, Vol. 10/5, p. e0126354, http://dx.doi.org/10.1371/journal.pone.0126354.
[109] Anderson, D. and J. Rense (2016), Harmful Algal Blooms Assessing Chile’s Historic HAB Events of 2016, A Report Prepared for the Global Aquaculture Alliance, https://www.aquaculturealliance.org/wp-content/uploads/2017/05/Final-Chile-report.pdf.
[20] Azevedo, A. et al. (2017), “An oil risk management system based on high-resolution hazard and vulnerability calculations”, Ocean & Coastal Management, Vol. 136, pp. 1-18, http://dx.doi.org/10.1016/J.OCECOAMAN.2016.11.014.
[124] Bastos Gomes, G. et al. (2017), “Use of environmental DNA (eDNA) and water quality data to predict protozoan parasites outbreaks in fish farms”, Aquaculture, Vol. 479, pp. 467-473, http://dx.doi.org/10.1016/J.AQUACULTURE.2017.06.021.
[87] Batista, W. et al. (2017), “Which Ballast Water Management System Will You Put Aboard? Remnant Anxieties: A Mini-Review”, Environments, Vol. 4/3, p. 54, http://dx.doi.org/10.3390/environments4030054.
[92] Bawat (2018), Ballast Water Management System for ships and vessels, https://www.bawat.com/ship-bwms/ship-bwms.
[77] Bento, N. and M. Fontes (2017), Direction and legitimation in system upscaling - planification of floating offshore wind, http://dx.doi.org/10.15847/dinamiacet-iul.wp.2017.01.
[170] Bergh, O. et al. (2017), Impact of global warming on diseases in aquaculture, European Aquaculture Society - Meeting Abstract, https://www.was.org/easonline/AbstractDetail.aspx?i=8583.
[162] Bergström, L. et al. (2014), “Effects of offshore wind farms on marine wildlife—a generalized impact assessment”, Environmental Research Letters, Vol. 9/3, p. 034012, http://dx.doi.org/10.1088/1748-9326/9/3/034012.
[111] Besson, M. et al. (2017), “Effect of production quotas on economic and environmental values of growth rate and feed efficiency in sea cage fish farming”, PLOS ONE, Vol. 12/3, p. e0173131, http://dx.doi.org/10.1371/journal.pone.0173131.
[168] Bjelland H. (2018), Current challenges and future opportunities for exposed salmon farming in Norway, presentation at Oceanology International 2018, https://www.arcticfrontiers.com/wp-content/uploads/downloads/2018/Arctic%20Frontiers%20Science/Presentations/23%20January%202018/Aquaculture%20in%20the%20High%20North%20in%20times%20of%20change/1500%20Bjelland_Hans.pdf (accessed on 5 December 2018).
[27] Boschen, R. et al. (2016), “A primer for use of genetic tools in selecting and testing the suitability of set-aside sites protected from deep-sea seafloor massive sulfide mining activities”, Ocean & Coastal Management, Vol. 122, pp. 37-48, http://dx.doi.org/10.1016/J.OCECOAMAN.2016.01.007.
[107] Bricker, S. et al. (2016), “Integration of ecosystem-based models into an existing interactive web-based tool for improved aquaculture decision-making”, Aquaculture, Vol. 453, pp. 135-146, http://dx.doi.org/10.1016/J.AQUACULTURE.2015.11.036.
[94] Brinkmeyer, R. (2016), “Diversity of bacteria in ships ballast water as revealed by next generation DNA sequencing”, Marine Pollution Bulletin, Vol. 107/1, pp. 277-285, http://dx.doi.org/10.1016/J.MARPOLBUL.2016.03.058.
[149] BSEE (2015), Rigs to Reefs | Bureau of Safety and Environmental Enforcement, https://www.bsee.gov/what-we-do/environmental-focuses/rigs-to-reefs.
[127] Business Wire (2016), Global Medicines for Aquaculture Market Report 2016 - Analysis, Technologies & Forecasts - Research and Markets | Business Wire, https://www.businesswire.com/news/home/20160627006068/en/Global-Medicines-Aquaculture-Market-Report-2016.
[76] Carbon Trust and Offshore Renewable Energy Catapult (2017), Floating Wind Joint Industry Project: Policy and Regulatory Appraisal, https://www.carbontrust.com/media/673978/wp1-flw-jip-policy-regulatory-appraisal_final_170120_clean.pdf (accessed on 5 December 2018).
[62] Catapult and Carbon Trust (2017), Floating Wind Joint Industry Project: Policy and Regulatory Appraisal, Carbon Trust and Catapult Offshore Renewable Energy, https://www.carbontrust.com/media/673978/wp1-flw-jip-policy-regulatory-appraisal_final_170120_clean.pdf.
[36] Chardard, Y. (2017), UTOFIA – A novel solution to underwater 3D real time imaging using range gating technology.
[140] Cimino, R. (2017), Decommissioning infrastructures at sea: reducing environmental impact; enhancing blue growth opportunities.
[151] Claisse, J. et al. (2014), “Oil platforms off California are among the most productive marine fish habitats globally.”, Proceedings of the National Academy of Sciences of the United States of America, Vol. 111/43, pp. 15462-7, http://dx.doi.org/10.1073/pnas.1411477111.
[98] Clarksons Research (2017), Ballast Water Management Update, http://www.clarksons.net/docdata/public/newsdownloads/bwms_update.pdf.
[37] Colefax, A., P. Butcher and B. Kelaher (2018), “The potential for unmanned aerial vehicles (UAVs) to conduct marine fauna surveys in place of manned aircraft”, ICES Journal of Marine Science, Vol. 75/1, pp. 1-8, http://dx.doi.org/10.1093/icesjms/fsx100.
[110] Copernicus (2016), Forecasting Harmful Algal Blooms for fish and shellfish farmers The ASIMUTH project, http://ec.europa.eu/fisheries/documentation/publications/2015-aquaculture-facts_en.pdf.
[1] Council of Canadian Academies (2013), Ocean Science in Canada: Meeting the Challenge, Seizing the Opportunity, Expert Panel on Canadian Ocean Science, Ottawa, https://www.scienceadvice.ca/wp-content/uploads/2018/10/oceans_fullreporten.pdf.
[152] Cresson, P., S. Ruitton and M. Harmelin-Vivien (2014), “Artificial reefs do increase secondary biomass production: mechanisms evidenced by stable isotopes”, Marine Ecology Progress Series, Vol. 509, pp. 15-26, http://dx.doi.org/10.3354/meps10866.
[33] Cutter, G., K. Stierhoff and D. Demer (n.d.), “Remote sensing of habitat characteristics using echo metrics and image-based seabed classes”, http://dx.doi.org/10.1093/icesjms/fsw024.
[126] Dadar, M. et al. (2017), “Advances in Aquaculture Vaccines Against Fish Pathogens: Global Status and Current Trends”, Reviews in Fisheries Science & Aquaculture, Vol. 25/3, pp. 184-217, http://dx.doi.org/10.1080/23308249.2016.1261277.
[26] Darling, J. et al. (2017), “Recommendations for developing and applying genetic tools to assess and manage biological invasions in marine ecosystems”, Marine Policy, Vol. 85, pp. 54-64, http://dx.doi.org/10.1016/J.MARPOL.2017.08.014.
[90] Davidson, I. et al. (2017), “Pioneering patterns of ballast treatment in the emerging era of marine vector management”, Marine Policy, Vol. 78, pp. 158-162, http://dx.doi.org/10.1016/J.MARPOL.2017.01.021.
[86] Davidson, K. et al. (2016), “Forecasting the risk of harmful algal blooms”, Harmful Algae, Vol. 53, pp. 1-7, http://dx.doi.org/10.1016/j.hal.2015.11.005.
[131] Defoirdt, T., P. Sorgeloos and P. Bossier (2011), “Alternatives to antibiotics for the control of bacterial disease in aquaculture”, Current Opinion in Microbiology, Vol. 14/3, pp. 251-258, http://dx.doi.org/10.1016/j.mib.2011.03.004.
[161] Department of Industry of the Australian Government (2018), Offshore Petroleum Decommissioning Guideline, http://www.nopta.gov.au.
[4] Division for Ocean Affairs and the Law of the Sea, O. (ed.) (2017), The First Global Integrated Marine Assessment, Cambridge University Press, Cambridge, http://dx.doi.org/10.1017/9781108186148.
[39] Dooly, G. et al. (2016), “Unmanned vehicles for maritime spill response case study: Exercise Cathach”, Marine Pollution Bulletin, Vol. 110/1, pp. 528-538, http://dx.doi.org/10.1016/j.marpolbul.2016.02.072.
[118] Dossou, S. et al. (2018), “Effect of partial replacement of fish meal by fermented rapeseed meal on growth, immune response and oxidative condition of red sea bream juvenile, Pagrus major”, Aquaculture, Vol. 490, pp. 228-235, http://dx.doi.org/10.1016/J.AQUACULTURE.2018.02.010.
[64] Dvorak, P. (2018), Riding the waves of complexity in floating offshore wind, https://www.windpowerengineering.com/projects/offshore-wind/riding-the-waves-of-complexity-in-floating-offshore-wind/.
[3] European Marine Board (2013), Navigating the Future, Position Paper 20, March, http://www.marineboard.eu.
[101] FAO (2018), The State of World Fisheries and Aquaculture: Meeting the Sustainable Development Goals, http://www.fao.org/publications.
[114] FAO (2017), “FAO Aquaculture Newsletter”, Vol. No. 56/April, http://www.fao.org/3/a-i7171e.pdf.
[95] Fernandes, J. et al. (2016), “Costs and benefits to European shipping of ballast-water and hull-fouling treatment: Impacts of native and non-indigenous species”, Marine Policy, Vol. 64, pp. 148-155, http://dx.doi.org/10.1016/J.MARPOL.2015.11.015.
[85] Fernandes, J. et al. (2016), “Costs and benefits to European shipping of ballast-water and hull-fouling treatment: Impacts of native and non-indigenous species”, Marine Policy, Vol. 64, pp. 148-155, http://dx.doi.org/10.1016/J.MARPOL.2015.11.015.
[48] Fernandez-Ordonez, Y., J. Soria-Ruiz and C. Medina-Ramirez (2015), Use of satellite image data to locate potential aquaculture facilities, https://www.researchgate.net/publication/304154730_Use_of_satellite_image_data_to_locate_potential_aquaculture_facilities.
[104] Føre, M. et al. (2018), “Precision fish farming: A new framework to improve production in aquaculture”, Biosystems Engineering, Vol. 173, pp. 176-193, http://dx.doi.org/10.1016/j.biosystemseng.2017.10.014.
[38] Forshaw, K. (2018), Linking Ocean Sustainability and Innovation.
[147] Fowler, A. et al. (2018), “Environmental benefits of leaving offshore infrastructure in the ocean”, Frontiers in Ecology and the Environment, Vol. 16/10, pp. 571-578, http://dx.doi.org/10.1002/fee.1827.
[72] Fraunhofer (2016), Long-lasting rust protection for offshore wind turbines, https://www.fraunhofer.de/en/press/research-news/2016/February/long-lasting-rust-protection-for-offshore-wind-turbines.html.
[135] Froehlich, H. et al. (2017), “Offshore Aquaculture: I Know It When I See It”, Frontiers in Marine Science, Vol. 4, p. 154, http://dx.doi.org/10.3389/fmars.2017.00154.
[55] Gallego, A. et al. (2017), “Large scale three-dimensional modelling for wave and tidal energy resource and environmental impact: Methodologies for quantifying acceptable thresholds for sustainable exploitation”, Ocean & Coastal Management, Vol. 147, pp. 67-77, http://dx.doi.org/10.1016/j.ocecoaman.2016.11.025.
[40] Gates, A. (2018), Interspill 2018 Application of Marine Autonomous Systems to oil spill response and monitoring Conference Stream: Surveillance, Modelling and Visualisation (SMV), http://www.interspill.org/previous-events/2018/14March2018/3-Situational-Awareness/Application-of-Marine-Autonomous-Systems-A-Gates-NOC-and-S-Hall-OSRL.pdf.
[133] Gentry, R. et al. (2017), “Mapping the global potential for marine aquaculture”, Nature Ecology & Evolution, Vol. 1/9, pp. 1317-1324, http://dx.doi.org/10.1038/s41559-017-0257-9.
[30] Geoffroy, M. et al. (2016), “AUV-based acoustic observations of the distribution and patchiness of pelagic scattering layers during midnight sun”, ICES Journal of Marine Science: Journal du Conseil, Vol. 74/9, p. fsw158, http://dx.doi.org/10.1093/icesjms/fsw158.
[23] Gomez, F. et al. (2017), “Intraseasonal patterns in coastal plankton biomass off central Chile derived from satellite observations and a biochemical model-NC-ND license (http:// creativecommons.org/licenses/by-nc-nd/4.0/)”, http://dx.doi.org/10.1016/j.jmarsys.2017.05.003.
[148] Grasso, M. (2017), Rigs to Reefs Program.
[129] Grave, K. and E. Oslo (2016), Report Use of Antibiotics in Norwegian Aquaculture Report from The Norwegian Veterinary Institute Use of Antibiotics in Norwegian Aquaculture on behalf of Norwegian Seafood Council, https://seafood.no/contentassets/c5a14b9acf3b4f1b9753263586513a68/use-of-antibiotics-in-norwegian-aquaculture.pdf.
[136] Grealis, E. et al. (2017), “The economic impact of aquaculture expansion: An input-output approach”, Marine Policy, Vol. 81, pp. 29-36, http://dx.doi.org/10.1016/J.MARPOL.2017.03.014.
[21] Guichoux, Y. (2018), Omni-situ surface currents: a transformative approach to measure ocean surface currents using AIS data.
[66] GWEC Global Wind Energy Council (2017), Global wind report: Opening up new markets for business, http://www.gwec.net.
[54] Heath, M. et al. (2017), “Modelling the sensitivity of suspended sediment profiles to tidal current and wave conditions”, Ocean & Coastal Management, Vol. 147, pp. 49-66, http://dx.doi.org/10.1016/J.OCECOAMAN.2016.10.018.
[160] Henry, L. et al. (2017), ANChor Summary Report Final 12/17, https://s3-eu-west-1.amazonaws.com/static.insitenorthsea.org/files/INSITE-ANChor-Summary-Report-v1.0.pdf.
[117] Henry, M. et al. (2015), “Review on the use of insects in the diet of farmed fish: Past and future”, Animal Feed Science and Technology, Vol. 203, pp. 1-22, http://dx.doi.org/10.1016/J.ANIFEEDSCI.2015.03.001.
[63] Hill, J. (2018), Hywind Scotland, World’s First Floating Wind Farm, Performing Better Than Expected | CleanTechnica, https://cleantechnica.com/2018/02/16/hywind-scotland-worlds-first-floating-wind-farm-performing-better-expected/.
[79] Höfling, H. (2016), “Cost of Renewable energy – how expensive is green electricity really?”, Fokus Volkswirtschaft, Vol. 45/6 October 2015.
[172] Hukkelas, T. (2018), Ocean Farm 1: The world’s first “smart” fish farm.
[81] Hurley, W. (2018), The fundamental importance of mooring systems, http://www.rechargenews.com.
[59] IEA (2018), Renewables 2018: Analysis and Forecasts to 2023, IEA, Paris, https://dx.doi.org/10.1787/re_mar-2018-en.
[7] IEA (2018), The future of petrochemicals: Towards more sustainable plastics and fertilisers, IEA, Paris, https://dx.doi.org/10.1787/9789264307414-en.
[141] IHS Markit (2016), Decommissioning of Aging Offshore Oil and Gas Facilities Increasing Significantly, with Annual Spending Rising to $13 Billion by 2040, IHS Markit Says | IHS Online Newsroom, https://news.ihsmarkit.com/press-release/energy-power-media/decommissioning-aging-offshore-oil-and-gas-facilities-increasing-si.
[84] IMO (2018), Invasive Aquatic Species (IAS), http://www.imo.org/en/OurWork/Environment/BallastWaterManagement/Pages/AquaticInvasiveSpecies(AIS).aspx.
[88] IMO (2017), Global treaty to halt invasive aquatic species enters into force, Briefing, http://www.imo.org/en/MediaCentre/PressBriefings/Pages/21-BWM-EIF.aspx.
[103] Innes, J., R. Martini and A. Leroy (2017), “Red tape and administrative burden in aquaculture licensing”, OECD Food, Agriculture and Fisheries Papers, No. 107, OECD Publishing, Paris, https://dx.doi.org/10.1787/7a56bfbc-en.
[159] INSITE International Scientific Advisory Board ISAB (2018), The Influence of Man-made Structures in the North Sea (INSITE) Synthesis and Assessment of Phase 1, https://s3-eu-west-1.amazonaws.com/static.insitenorthsea.org/files/INSITE-ISAB-Synthesis-Report-Phase-1-final.pdf.
[139] International Energy Agency (2018), Offshore Energy Outlook, http://www.iea.org/t&c/.
[41] International Transport Forum (2018), Data sharing in the maritime logistics chain (draft paper).
[10] IOC (2018), Roadmap for the UN Decade of Ocean Science for Sustainable Development, Intergovernmental Oceanographic Commission (IOC) , Fifty-first Session of the Executive Council UNESCO, Paris, 3–6 July 2018.
[6] IOC (2017), Global Ocean Science Report : The Current Status of Ocean Science Around the World, , Intergovernmental Oceanographic Commission, UNESCO Publishing, https://unesdoc.unesco.org/ark:/48223/pf0000250428.
[9] IPCC (2018), The Ocean and Cryosphere in a Changing Climate, Special Report Webpage, https://www.ipcc.ch/report/srocc/ (accessed on 16 June 2018).
[138] Jagerroos, S. and P. R Krause (2016), “Rigs-To-Reef; Impact or Enhancement on Marine Biodiversity”, Journal of Ecosystem & Ecography, Vol. 6/2, pp. 1-9, http://dx.doi.org/10.4172/2157-7625.1000187.
[69] James, E., S. Benjamin and M. Marquis (2018), “Offshore wind speed estimates from a high-resolution rapidly updating numerical weather prediction model forecast dataset”, Wind Energy, Vol. 21/4, pp. 264-284, http://dx.doi.org/10.1002/we.2161.
[47] Jha, S. et al. (2017), “Renewable energy: Present research and future scope of Artificial Intelligence”, Renewable and Sustainable Energy Reviews, Vol. 77, pp. 297-317, http://dx.doi.org/10.1016/j.rser.2017.04.018.
[105] Joffre, O. et al. (2017), “How is innovation in aquaculture conceptualized and managed? A systematic literature review and reflection framework to inform analysis and action”, Aquaculture, Vol. 470, pp. 129-148, http://dx.doi.org/10.1016/J.AQUACULTURE.2016.12.020.
[153] Jørgensen, D. (2012), “OSPAR’s exclusion of rigs-to-reefs in the North Sea”, Ocean & Coastal Management, Vol. 58, pp. 57-61, http://dx.doi.org/10.1016/j.ocecoaman.2011.12.012.
[61] Kadiyala, A., R. Kommalapati and Z. Huque (2017), “Characterization of the life cycle greenhouse gas emissions from wind electricity generation systems”, International Journal of Energy and Environmental Engineering, Vol. 8/1, pp. 55-64, http://dx.doi.org/10.1007/s40095-016-0221-5.
[73] Kausche, M. et al. (2018), “Floating offshore wind - Economic and ecological challenges of a TLP solution”, Renewable Energy, Vol. 126, pp. 270-280, http://dx.doi.org/10.1016/J.RENENE.2018.03.058.
[173] Kelly, D. (2018), InnovaSea: phased development of an open ocean aquaculture farm.
[24] Kim, H. et al. (2017), “Remote sensing and water quality indicators in the Korean West coast: Spatio-temporal structures of MODIS-derived chlorophyll-a and total suspended solids”, Marine Pollution Bulletin, Vol. 121/1-2, pp. 425-434, http://dx.doi.org/10.1016/j.marpolbul.2017.05.026.
[96] King D.M. et al. (2012), “(PDF) Preview of global ballast water treatment markets”, Journal of Marine Engineering, Science and Technology, Vol. 11/1, pp. 3-15, https://www.researchgate.net/publication/290997328_Preview_of_global_ballast_water_treatment_markets.
[83] King, D. (2016), Ocean Health and the Economics of Global Ballast Water Regulations, International Network for Environmental Compliance and Enforcement (INECE), https://inece.org/library/.
[123] Kleitou, P., D. Kletou and J. David (2018), “Is Europe ready for integrated multi-trophic aquaculture? A survey on the perspectives of European farmers and scientists with IMTA experience”, Aquaculture, Vol. 490, pp. 136-148, http://dx.doi.org/10.1016/J.AQUACULTURE.2018.02.035.
[112] Komen, H. et al. (2017), “Impact of selective breeding on European aquaculture”, Aquaculture, Vol. 472, pp. 8-16, http://dx.doi.org/10.1016/j.aquaculture.2016.03.012.
[46] Kongsberg (2017), Underwater Science Products: Technology for Sustainable Fisheries.
[22] Kroodsma, D. et al. (2018), “Tracking the global footprint of fisheries”, Science, Vol. 359/6378, pp. 904-908, http://dx.doi.org/10.1126/science.aao5646.
[50] Kubryakov, A. et al. (2018), “Reconstructing Large- and Mesoscale Dynamics in the Black Sea Region from Satellite Imagery and Altimetry Data—A Comparison of Two Methods”, Remote Sensing, Vol. 10/2, p. 239, http://dx.doi.org/10.3390/rs10020239.
[57] Kulkarni, S., M. Deo and S. Ghosh (2018), “Framework for assessment of climate change impact on offshore wind energy”, Meteorological Applications, Vol. 25/1, pp. 94-104, http://dx.doi.org/10.1002/met.1673.
[70] Kulkarni, S., M. Deo and S. Ghosh (2018), “Framework for assessment of climate change impact on offshore wind energy”, Meteorological Applications, Vol. 25/1, pp. 94-104, http://dx.doi.org/10.1002/met.1673.
[58] Lader, P. et al. (2017), Classification of Aquaculture Locations in Norway With Respect to Wind Wave Exposure, ASME, http://dx.doi.org/10.1115/OMAE2017-61659.
[89] Latarche, M. (2017), “What ballast water technologies are available?”, ShipInsight 10 April 2017, https://shipinsight.com/what-ballast-water-technologies-are-available.
[167] Leber, J. (2018), Global Insurance Industry Steps Up to Turn Ocean Risk Into — Oceans Deeply, https://www.newsdeeply.com/oceans/articles/2018/05/15/global-insurance-industry-steps-up-to-turn-ocean-risk-into-resilience.
[97] Linder M. (2017), Economic Impact of Global Standards - Ballast Water Treatment, http://injapan.no/wp-content/uploads/2017/01/7-Mr.-Martin-Linder-Optimarin-Ballast-Water-Treatment.pdf (accessed on 5 December 2018).
[13] Li, P. et al. (2016), “Offshore oil spill response practices and emerging challenges”, Marine Pollution Bulletin, Vol. 110/1, pp. 6-27, http://dx.doi.org/10.1016/J.MARPOLBUL.2016.06.020.
[128] López-Cortés, X. et al. (2017), “Fast detection of pathogens in salmon farming industry”, Aquaculture, Vol. 470, pp. 17-24, http://dx.doi.org/10.1016/J.AQUACULTURE.2016.12.008.
[157] Lyons, Y. et al. (2013), International Workshop on Rigs-toReefs: Prospects for large artificial reefs in tropical South East Asia – Is there life after oil?, https://cil.nus.edu.sg/wp-content/uploads/2015/03/Executive-Summary.pdf.
[29] Martignac, F. et al. (2015), “The use of acoustic cameras in shallow waters: new hydroacoustic tools for monitoring migratory fish population. A review of DIDSON technology”, Fish and Fisheries, Vol. 16/3, pp. 486-510, http://dx.doi.org/10.1111/faf.12071.
[93] Maw, M. et al. (2018), “A Changeable Lab-on-a-Chip Detector for Marine Nonindigenous Microorganisms in Ship’s Ballast Water”, Micromachines, Vol. 9/1, p. 20, http://dx.doi.org/10.3390/mi9010020.
[71] McKenna, R., P. Ostman v.d. Leye and W. Fichtner (2016), “Key challenges and prospects for large wind turbines”, Renewable and Sustainable Energy Reviews, Vol. 53, pp. 1212-1221, http://dx.doi.org/10.1016/J.RSER.2015.09.080.
[51] Miller, P. (2018), Satellite based harmful algal bloom and water quality monitoring for coastal and offshore aquaculture farms to support management decisions.
[165] Multiconsult (2011), “Etterlating offshore og disponering ved land”, Report for the Norwegian Directorate of Climate and Pollution.
[19] Mussells, O., J. Dawson and S. Howell (2017), “Navigating pressured ice: Risks and hazards for winter resource-based shipping in the Canadian Arctic”, Ocean & Coastal Management, Vol. 137, pp. 57-67, http://dx.doi.org/10.1016/J.OCECOAMAN.2016.12.010.
[78] Myhr, A. et al. (2014), “Levelised cost of energy for offshore floating wind turbines in a life cycle perspective”, Renewable Energy, Vol. 66, pp. 714-728, http://dx.doi.org/10.1016/J.RENENE.2014.01.017.
[2] National Research Council (2015), Sea Change: 2015-2025 Decadal Survey of Ocean Sciences, National Academies Press, Washington, DC, http://dx.doi.org/10.17226/21655.
[18] Nevalainen, M., I. Helle and J. Vanhatalo (2017), “Preparing for the unprecedented — Towards quantitative oil risk assessment in the Arctic marine areas”, Marine Pollution Bulletin, Vol. 114/1, pp. 90-101, http://dx.doi.org/10.1016/J.MARPOLBUL.2016.08.064.
[125] Norris, A. (2017), “Application of genomics in salmon aquaculture breeding programs by Ashie Norris”, Marine Genomics, Vol. 36, pp. 13-15, http://dx.doi.org/10.1016/j.margen.2017.11.013.
[155] Norsk Petroleum (2018), Cessation and decommissioning - Norwegianpetroleum.no, https://www.norskpetroleum.no/en/developments-and-operations/cessation-and-decommissioning/.
[132] Norwegian Directorate of Fisheries (2018), Cleanerfish (Lumpfish and Wrasse), https://www.fiskeridir.no/English/Aquaculture/Statistics/Cleanerfish-Lumpfish-and-Wrasse.
[8] OECD (2018), Improving Markets for Recycled Plastics: Trends, Prospects and Policy Responses, OECD Publishing, Paris, https://dx.doi.org/10.1787/9789264301016-en.
[99] OECD (2017), Analysis of selected measures promoting the construction and operation of greener ships, OECD, Paris, http://www.oecd.org/sti/shipbuilding.
[100] OECD (2017), Analysis of Selected Measures Promoting the Construction and Operation of Greener Ships, OECD, Paris.
[11] OECD (2016), The Ocean Economy in 2030, OECD Publishing, Paris, https://dx.doi.org/10.1787/9789264251724-en.
[102] OECD/FAO (2018), OECD-FAO Agricultural Outlook 2018-2027, OECD Publishing, Paris/FAO, Rome, https://dx.doi.org/10.1787/agr_outlook-2018-en.
[166] Offshore Digital Magazine (2017), Xodus, Subcon form ‘rigs to reef’ decommissioning partnership - Offshore, https://www.offshore-mag.com/articles/2017/11/xodus-subcon-form-rigs-to-reef-decommissioning-partnership.html.
[65] offshoreWIND.biz (2017), BNEF: 237MW of Floating Offshore Wind by 2020 | Offshore Wind, https://www.offshorewind.biz/2017/03/20/bnef-237mw-of-floating-offshore-wind-by-2020/.
[137] OFS Research/Westwood Global Energy Group (2018), World Offshore Maintenance, Modifications & Operations 2018-2022, https://www.westwoodenergy.com/product/world-offshore-maintenance-modifications-operations-market-forecast-2018-2022/?utm_source=iContact&utm_medium=email&utm_campaign=2_OFS+Research&utm_content=.
[146] Osmundsen, P. and R. Tveterås (2003), “Decommissioning of petroleum installations—major policy issues”, Energy Policy, Vol. 31/15, pp. 1579-1588, http://dx.doi.org/10.1016/S0301-4215(02)00224-0.
[106] Ottinger, M., K. Clauss and C. Kuenzer (2016), “Aquaculture: Relevance, distribution, impacts and spatial assessments – A review”, Ocean & Coastal Management, Vol. 119, pp. 244-266, http://dx.doi.org/10.1016/J.OCECOAMAN.2015.10.015.
[134] Oyinlola, M. et al. (2018), “Global estimation of areas with suitable environmental conditions for mariculture species”, PLOS ONE, Vol. 13/1, p. e0191086, http://dx.doi.org/10.1371/journal.pone.0191086.
[28] Pereira, N. et al. (2016), “Challenges to implementing a ballast water remote monitoring system”, Ocean & Coastal Management, Vol. 131, pp. 25-38, http://dx.doi.org/10.1016/J.OCECOAMAN.2016.07.008.
[116] Perez-Velazquez, M. et al. (2018), “Partial replacement of fishmeal and fish oil by algal meals in diets of red drum Sciaenops ocellatus”, Aquaculture, Vol. 487, pp. 41-50, http://dx.doi.org/10.1016/J.AQUACULTURE.2018.01.001.
[82] Pezy, J., A. Raoux and J. Dauvin (2018), “An ecosystem approach for studying the impact of offshore wind farms: a French case study”, ICES Journal of Marine Science, http://dx.doi.org/10.1093/icesjms/fsy125.
[154] Porritt, J. (2017), Decommissioning in the North Sea: Rigs, Reefs, but not much Realism | Jonathon Porritt, 20.02.2017, http://www.jonathonporritt.com/blog/decommissioning-north-sea-rigs-reefs-not-much-realism.
[164] Raoux, A. et al. (2017), “Benthic and fish aggregation inside an offshore wind farm: Which effects on the trophic web functioning?”, Ecological Indicators, Vol. 72, pp. 33-46, http://dx.doi.org/10.1016/j.ecolind.2016.07.037.
[67] Renewable Energy Agency, I. (2016), Floating Foundations: A Game Changer for Offshore Wind Power, https://www.irena.org/DocumentDownloads/Publications/IRENA_Offshore_Wind_Floating_Foundations_2016.pdf.
[122] Research and Markets (2017), Aquafeed Market by End User, Ingredient, Additive, and Region - Global Forecast to 2022, April, https://www.researchandmarkets.com/research/vlhxv6/aquafeed_market.
[169] SAIC Scottish Aquaculture Innovation Centre (2018), Innovation and the Ripple Effect, http://scottishaquaculture.com/innovation-and-the-ripple-effect/.
[74] SeaTwirl (2018), SeaTwirl is developing a floating wind turbine, VAWT, for the ocean., https://seatwirl.com/.
[31] Shafait, F. et al. (2016), “Fish identification from videos captured in uncontrolled underwater environments”, ICES Journal of Marine Science: Journal du Conseil, Vol. 73/10, pp. 2737-2746, http://dx.doi.org/10.1093/icesjms/fsw106.
[143] Shaw, J., P. Seares and S. Newman (2018), Decommissioning offshore infrastructure: a review of stakeholder views and science priorities, WAMSI, Perth, Western Australia, http://www.wamsi.org.au/decommissioning-offshore-infrastructure-review-stakeholder-views-and-science-priorities.
[115] Shepherd, C., O. Monroig and D. Tocher (2017), “Future availability of raw materials for salmon feeds and supply chain implications: The case of Scottish farmed salmon”, Aquaculture, Vol. 467, pp. 49-62, http://dx.doi.org/10.1016/j.aquaculture.2016.08.021.
[35] Siddiqui, S. et al. (2018), “Automatic fish species classification in underwater videos: exploiting pre-trained deep neural network models to compensate for limited labelled data”, ICES Journal of Marine Science, Vol. 75/1, pp. 374-389, http://dx.doi.org/10.1093/icesjms/fsx109.
[52] Side, J. et al. (2017), “Developing methodologies for large scale wave and tidal stream marine renewable energy extraction and its environmental impact: An overview of the TeraWatt project”, Ocean & Coastal Management, Vol. 147, pp. 1-5, http://dx.doi.org/10.1016/J.OCECOAMAN.2016.11.015.
[12] Singha, S. and R. Ressel (2016), “Offshore platform sourced pollution monitoring using space-borne fully polarimetric C and X band synthetic aperture radar”, Marine Pollution Bulletin, Vol. 112/1-2, pp. 327-340, http://dx.doi.org/10.1016/J.MARPOLBUL.2016.07.044.
[145] Slav, I. (2018), Asian Oil Companies Face $100B Well Decommissioning Bill | OilPrice.com, 1 February 2018, https://oilprice.com/Latest-Energy-News/World-News/Asian-Oil-Companies-Face-100B-Well-Decommissioning-Bill.html (accessed on 5 December 2018).
[163] Slavik, K. et al. (2018), The large-scale impact of offshore wind farm structures on pelagic primary productivity in the southern North Sea, Springer International Publishing, http://dx.doi.org/10.1007/s10750-018-3653-5.
[142] Smyth, K. et al. (2015), “Renewables-to-reefs? – Decommissioning options for the offshore wind power industry”, Marine Pollution Bulletin, Vol. 90/1-2, pp. 247-258, http://dx.doi.org/10.1016/J.MARPOLBUL.2014.10.045.
[17] Spaulding, M. (2017), “State of the art review and future directions in oil spill modeling”, Marine Pollution Bulletin, Vol. 115/1-2, pp. 7-19, http://dx.doi.org/10.1016/J.MARPOLBUL.2017.01.001.
[16] Strong, J. and M. Elliott (2017), “The value of remote sensing techniques in supporting effective extrapolation across multiple marine spatial scales”, Marine Pollution Bulletin, Vol. 116/1-2, pp. 405-419, http://dx.doi.org/10.1016/J.MARPOLBUL.2017.01.028.
[75] Taninoki Ryota, K. et al. (2017), “Dynamic Cable System for Floating Offshore Wind Power Generation”, SEI Technical Review, Vol. 84/April, pp. 53-58, http://www.abysse.co.jp/japan/index.html.
[156] Techera, E. and J. Chandler (2015), “Offshore installations, decommissioning and artificial reefs: Do current legal frameworks best serve the marine environment?”, Marine Policy, Vol. 59, pp. 53-60, http://dx.doi.org/10.1016/J.MARPOL.2015.04.021.
[130] Thanigaivel, S. et al. (2016), “Seaweeds as an alternative therapeutic source for aquatic disease management”, Aquaculture, Vol. 464, pp. 529-536, http://dx.doi.org/10.1016/j.aquaculture.2016.08.001.
[60] Thomson, C. and G. Harrison (2015), Life cycle costs and carbon emissions of wind power: Executive Summary Key Points, http://www.climatexchange.org.ukhttp://www.climatexchange.org.uk/index.php/download_file/554/http://www.climatexchange.org.uk/index.php/download_file/555/.
[15] Tornero, V. and G. Hanke (2016), “Chemical contaminants entering the marine environment from sea-based sources: A review with a focus on European seas”, Marine Pollution Bulletin, Vol. 112/1-2, pp. 17-38, http://dx.doi.org/10.1016/J.MARPOLBUL.2016.06.091.
[119] Torrecillas, S. et al. (2017), “Combined replacement of fishmeal and fish oil in European sea bass (Dicentrarchus labrax): Production performance, tissue composition and liver morphology”, Aquaculture, Vol. 474, pp. 101-112, http://dx.doi.org/10.1016/J.AQUACULTURE.2017.03.031.
[34] Trenkel, V., N. Handegard and T. Weber (2016), “Observing the ocean interior in support of integrated management”, ICES Journal of Marine Science: Journal du Conseil, Vol. 73/8, pp. 1947-1954, http://dx.doi.org/10.1093/icesjms/fsw132.
[158] UK Department for Business, E. (2018), Oil and gas: decommissioning of offshore installations and pipelines - GOV.UK, https://www.gov.uk/guidance/oil-and-gas-decommissioning-of-offshore-installations-and-pipelines.
[5] United Nations (2016), Summary of the First Global Integrated Marine Assessment, http://www.un.org/depts/los/global_reporting/WOA_RPROC/Summary.pdf.
[174] United States Environmental Portection Agency (2013), Impacts of Climate Change on the Occurrence of Harmful Algal Blooms, http://go.usa.gov/gYTH.
[53] Venugopal, V., R. Nemalidinne and A. Vögler (2017), “Numerical modelling of wave energy resources and assessment of wave energy extraction by large scale wave farms”, Ocean & Coastal Management, Vol. 147, pp. 37-48, http://dx.doi.org/10.1016/J.OCECOAMAN.2017.03.012.
[32] Wall, C., J. Jech and S. McLean (2016), “Increasing the accessibility of acoustic data through global access and imagery”, ICES Journal of Marine Science: Journal du Conseil, Vol. 73/8, pp. 2093-2103, http://dx.doi.org/10.1093/icesjms/fsw014.
[108] Wells, M. et al. (2015), Harmful algal blooms and climate change: Learning from the past and present to forecast the future, Elsevier, http://dx.doi.org/10.1016/J.HAL.2015.07.009.
[144] Westwood Global Energy Group (2018), Westwood Insight: Decommissioning – Challenge, Accepted! - Westwood Global Energy Group, January, https://www.westwoodenergy.com/news/westwood-insight/westwood-insight-decommissioning-challenge-accepted/.
[43] Westwood Global Energy Group (2018), Westwood Insight: Decommissioning – Challenge, Accepted! - Westwood Global Energy Group, https://www.westwoodenergy.com/news/westwood-insight/westwood-insight-decommissioning-challenge-accepted/.
[44] Westwood Global Energy Group (2018), World AUV Market Forecast 2018-2022 - Westwood, June, https://www.westwoodenergy.com/product/world-auv-market-forecast-2018-2022/.
[14] White, H. et al. (2016), “Methods of Oil Detection in Response to the Deepwater Horizon Oil Spill”, Oceanography, Vol. 29/3, pp. 76-87, http://dx.doi.org/10.5670/oceanog.2016.72.
[42] Wilby, B. (2016), “AUVs gain momentum in oil and gas operations”, Society of Petroleum Engineers, Vol. 5/5.
[68] Wind Europe (2017), Floating Offshore Wind Vision Statement, https://windeurope.org/wp-content/uploads/files/about-wind/reports/Floating-offshore-statement.pdf.
[80] Wiser, R. et al. (2016), Forecasting Wind Energy Costs and Cost Drivers: The Views of the World’s Leading Experts, http://eta-publications.lbl.gov/sites/default/files/lbnl-1005717.pdf.
[91] Wollenhaupt, G. (2017), ABS: Nearly half of ballast water systems ‘inoperable’ or ‘problematic’ - Professional Mariner - December/January 2018, Professional Mariner, http://www.professionalmariner.com/December-January-2018/Ballast-water-systems-inoperable-or-problematic/ (accessed on 5 December 2018).
[25] Wu, W., Y. Zhou and B. Tian (2017), “Coastal wetlands facing climate change and anthropogenic activities: A remote sensing analysis and modelling application”, Ocean & Coastal Management, Vol. 138, pp. 1-10, http://dx.doi.org/10.1016/J.OCECOAMAN.2017.01.005.
[120] Yıldız, M. et al. (2018), “The effects of fish oil replacement by vegetable oils on growth performance and fatty acid profile of rainbow trout: Re-feeding with fish oil finishing diet improved the fatty acid composition”, Aquaculture, Vol. 488, pp. 123-133, http://dx.doi.org/10.1016/J.AQUACULTURE.2017.12.030.
[121] Ytrestøyl, T., T. Aas and T. Åsgård (2015), “Utilisation of feed resources in production of Atlantic salmon (Salmo salar) in Norway”, Aquaculture, Vol. 448, pp. 365-374, http://dx.doi.org/10.1016/J.AQUACULTURE.2015.06.023.
[113] Yue, G. and L. Wang (2017), “Current status of genome sequencing and its applications in aquaculture”, Aquaculture, Vol. 468, pp. 337-347, http://dx.doi.org/10.1016/J.AQUACULTURE.2016.10.036.
[45] Zborowski, M. (2018), Total, Google Cloud to Team on AI for Upstream, 26 April 2018, https://www.spe.org/en/print-article/?art=4144.
[49] Zecchetto, S., Zecchetto and Stefano (2018), “Wind Direction Extraction from SAR in Coastal Areas”, Remote Sensing, Vol. 10/2, p. 261, http://dx.doi.org/10.3390/rs10020261.
[56] Zheng, C. et al. (2016), “An overview of global ocean wind energy resource evaluations”, Renewable and Sustainable Energy Reviews, Vol. 53, pp. 1240-1251, http://dx.doi.org/10.1016/J.RSER.2015.09.063.
Notes
← 1. Advances in other technologies, e.g. drone technology, could also contribute to reduced inspection costs.
← 2. Significant moves are also being made in bringing salmon farming onshore with the help of advances in such techniques as land-based Recycling Aquaculture Systems. These technologies are not addressed in this report since its focus is specifically on production in the marine environment.
← 3. Much of the current evidence suggests that under a number of climate change scenarios, harmful algal bloom incidents may increase. However, more research into the link between climate change and harmful algae is required. See for example (United States Environmental Portection Agency, 2013[174]).
← 4. It is worth noting that genetically modified salmon is already on the marketplace and is being presented as one of the solutions needed to make land-based salmon farming profitable (e.g. AquaBounty’s AquaAdvantage salmon, where a Chinook growth hormone gene has been integrated into the genome of an Atlantic salmon).
← 5. In South America, infectious salmon amenia cut the salmon harvest in Chile by 60% between 2008 and 2010. Chile was also at the centre of massive outbreaks of sea lice in 2007 which caused economic losses in the order of USD 2 billion (Ottinger, Clauss and Kuenzer, 2016[106]). In Europe, too, sea lice is proving a persistent challenge – for example, in 2011 they are estimated to have caused production losses of around USD 436 million, equivalent to 9% of total revenues of Norwegian fish farmers (Abolofia, Asche and Wilen, 2017[171]). In Asia, among the most potent diseases are the Whitespot Syndrome Virus and the Yellowhead Virus, which have triggered crop losses in shrimp farming running into millions of USD – in the mid-1990s the Whitespot Syndrome Virus was responsible for losses in Bangladesh of almost 45% of total shrimp production. Other cases of catastrophic disease outbreaks have been reported from Thailand, Vietnam, Peru, Nicaragua and Taiwan (Ottinger, Clauss and Kuenzer, 2016[106]). Climate change is set to complicate matters yet further. In Northern European waters, for example, rising ocean temperatures over the longer term are likely to lead to changes in the panorama of diseases affecting finfish, rendering them less vulnerable to some harmful viruses and bacteria but more vulnerable to others. In the case of sea lice, warmer water is likely to lead to increased infestations of the parasite (Bergh et al., 2017[170])
← 6. Initially, wild cleaner fish were used, but the resultant growing pressure on wild stocks of the species plus the overriding need to deal with recurring sea lice outbreaks led the salmon farming community to take action by developing cleaner-fish aquaculture. Whereas in 2012 farmed cleaner fish accounted for only a minute fraction of total wrasse and lumpfish use, the share had grown to 44% by 2016 (Norwegian Directorate for Fisheries). Over the same period the number of companies licensed to sell farmed cleaner fish rose from 5 to 24, and the value of those sales from NOK 7 million (about EUR 1 million) to NOK 304 million (about EUR 33 million). The total value of wild and farmed cleaner fish together was estimated at NOK 652 million (about EUR 70 million) in 2016. Research and development in cleaner fish has accelerated remarkably, and projects are currently running in Scotland, Ireland, Faroe Islands and Iceland. The Scottish Aquaculture Innovation Centre, for example, is collaborating on several projects aimed at inter alia scaling up the use of cleaner fish, improving cleaner fish vaccination, ensuring the sustainable supply and deployment of lumpsuckers, and enhancing their health and welfare (SAIC Scottish Aquaculture Innovation Centre, 2018[169]).
← 7. Alone in Norway there are currently 104 applications under way for innovation development licenses, ranging from coastal closed systems, to vessel re-use and long-ship type structures with no bottom (Bjelland H., 2018[168]).
← 8. It is a huge semi-submersible structure, anchored to the sea bed and suitable for water depths of 100 to 300 m, and big enough to produce1.6 million salmon of 5 kg in weight. The highly sophisticated technology is closely aligned to the concept of precision fish farming (Føre et al., 2018[104]). It brings together marine engineering, marine cybernetics and marine biology via a “big data” approach. The structure combines innovations from different parts of the enterprise and from various sectors. For example, its extensive subsea sensor suite comes from the maritime business and contains highly sensitive echo sounders originally developed to detect oil and gas leaks but deployed here to detect fish feed pellets. Feeding is much more precise so as to leave a smaller environmental footprint, relying heavily on bio-cybernetics to model behaviour and mathematical modelling for analysis of the metabolism. Among the next objectives are improved situational awareness and visualisation of fish, both capabilities among the most needed for all autonomous systems (Hukkelas, 2018[172]).
← 9. This is a complete turnkey farm system that can be scaled up incrementally as learning from experience improves and capital investment becomes available. Feeding and monitoring are automated, the data being transmitted to shore and to service vessels (Kelly, 2018[173]).