Climate change is a global challenge threatening the foundations of human wellbeing. To limit negative impacts, most OECD countries aim for net-zero domestic greenhouse gas emissions by 2050. Deep and broad transformations of economies will be needed. Impacts, local conditions and vulnerabilities vary across territories and by degree of rurality. For example, within-country variation in emissions is larger than between countries. Most OECD countries still have regions with coal-fired electricity generation far removed from short-term net-zero-consistent benchmarks. Poorer cities and rural regions are more car-dependent, making them more sensitive to the costs of decarbonising transport. Further, employment at risk is modest but regionally concentrated. Therefore, there is a need for place-based and regionally-balanced policies aligned with national and global objectives, to address climate change, mitigation and adaptation. Moreover, climate change mitigation brings well-being benefits, beyond the protection of the climate, that arise locally and in the near term. These can be major motivators for local action as they can more than offset mitigation costs. Delaying action raises costs. Vulnerable communities require support.
OECD Regional Outlook 2021
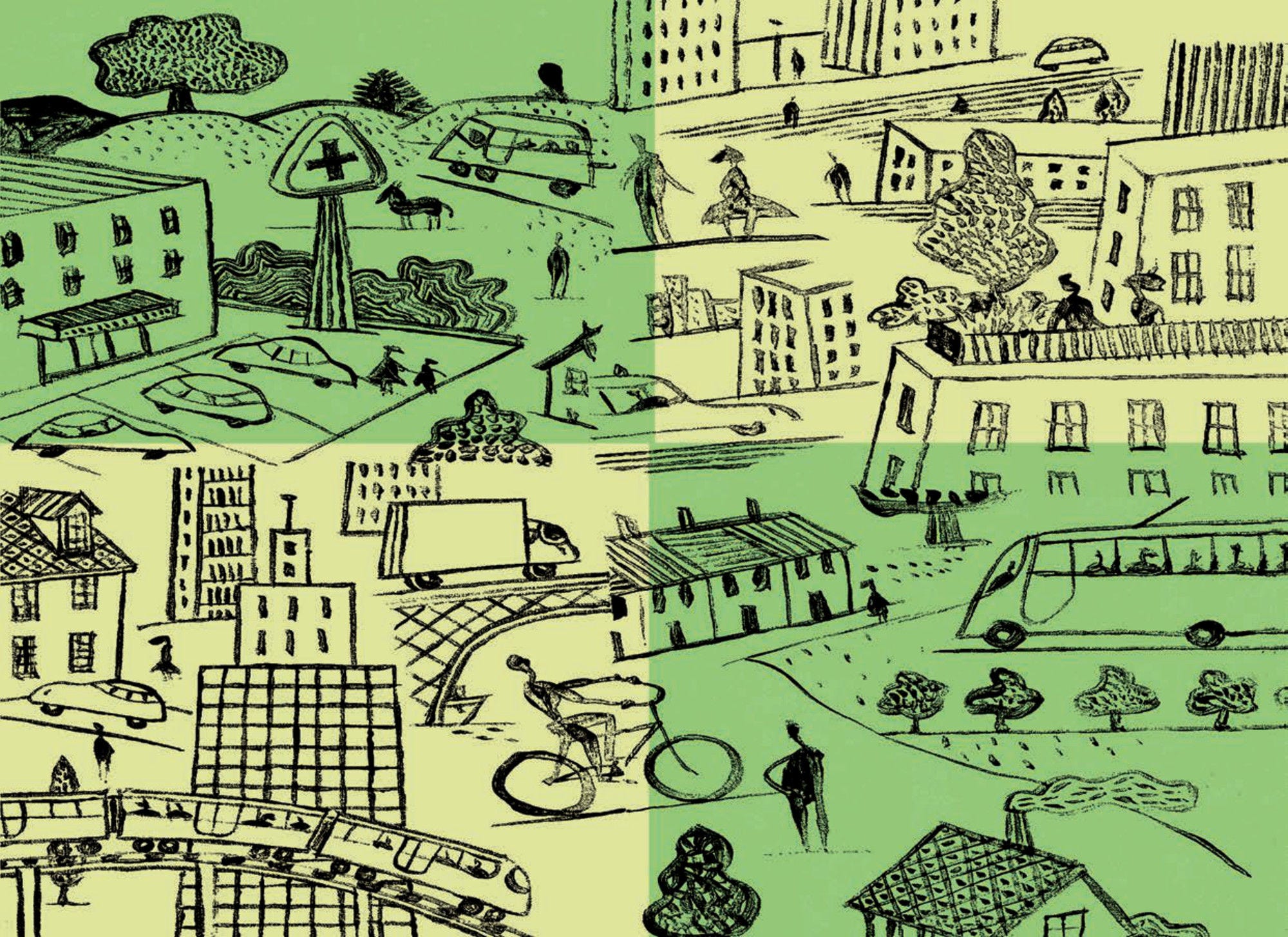
3. Reaching net-zero greenhouse gas emissions: The role for regions and cities
Abstract
The case for regional action
Addressing climate change is a global challenge requiring local action
OECD countries are increasingly recognising the urgent need to act on climate change, both to mitigate global warming and adapt to climate change which is already unavoidable. While these challenges are global, requiring multilateral and national action, they also require strong local actions and place-based policies that align with national and global objectives. As argued below, addressing challenges through a well-being perspective makes it easier and more rewarding for citizens and policymakers in regions and cities to integrate climate action in all decisions concerning place-based development. Doing so harnesses the multiple non-climate benefits and helps minimise trade-offs and vulnerabilities. These benefits can offset the net cost of climate action, often in full. Unlike the climate benefits themselves, many of these well-being gains arise locally and immediately and, therefore, decisively reinforce the case for integrating climate policy in regional development.
This Chapter sets out why climate policy and resulting wellbeing impacts are central to regional development policies, which is also developed in Accelerating Climate Action: Refocusing Policies through a Well-being Lens (2019[1]). The Chapter also includes a discussion of indicators to support evidence-based policies, building on existing OECD data that regions can already use to monitor progress as well as upcoming climate hazards. The following section in this chapter will discuss policies to move regional policymaking closer to achieving regional development in a way that is consistent with climate objectives.
Many OECD countries have set net-zero targets for their domestic greenhouse gas (GHG) emissions by 2050 or earlier. These include European Nordic countries, Canada, Korea, New Zealand (albeit excluding agricultural emissions and including international offsets), Switzerland, the United Kingdom (UK) and the European Union (albeit excluding aviation and shipping). They have done so recognising that high-income countries have a particular role to play in meeting the objective of the Paris agreement to limit global warming to well below 2 degrees and make efforts to limit it to 1.5°C (Climate Change Committee, 2019[2]). In addition, they will need to contribute to support emission reductions in poorer countries. Some emerging economies, including Chile and Costa Rica, have also set similar targets for their domestic emissions. Bhutan has already reached net-zero GHG emissions and its target is to keep it that way. Most recently, China has announced its aim to reach carbon neutrality by 2060. Overall, 126 countries have committed to climate or carbon neutrality by mid-century (Climate Analytics/New Climate Institute, n.d.[3]). Taken together, and assuming they are implemented, they may limit global warming to around 2.5°C by the end of the century. However, if human-made net CO2 emissions remain positive, they will continue to raise global average temperatures.
Reaching the objectives of the Paris Agreement will prevent major threats to the foundations of human well-being. These threats are substantially worse under 2°C than under 1.5°C. For example, key risks from 2°C and above for the use of land include worldwide food shortages, high impacts and risks of dryland water scarcity and large-scale wildfires in many regions (Figure 3.1). The very high risk and impact of permafrost degradation include the release of GHG (methane and CO2), in turn possibly further accelerating climate change (IPCC, 2018[4]). The Arctic ice sheet’s temperature sensitivity alone implies 1.3 metres of sea level rise per degree of warming up to 2°C above pre-industrial levels, almost doubling to 2.4 metres per degree of warming between 2°C and 6°C and increasing to approximately 10 metres per degree of warming between 6°C and 9°C. This implies very large sea level increases even within the Paris Agreement temperature limits and catastrophic increases beyond, though they would occur after the end of the 21st century (Garbe et al., 2020[5]). The experience of the COVID-19 crisis shows that risks to the foundations of human well-being, notably human health, can create systemic effects throughout the economy (Box 3.1). The emergence of new human diseases is also closely linked to the loss and degradation of ecosystems and habitats, which in turn is driven by climate change, resource extraction, urban and agricultural expansion and pollution (Rohr et al., 2019[6]). With a global temperature rise of 1.5°C, risks and impacts still include, for example, regional food insecurity. Limiting global warming to 1.5°C is likely unavoidable but feasible if rapid action is taken.
The later the peak of emissions is reached, the faster and bigger emission reductions will be necessary worldwide since it is the stock of cumulated CO2 that counts. This risk is aggravated as incremental global warming can trigger an increasing number of “tipping points”. These are climate-change-induced events that irreversibly feed back to global warming, such as the melting of the West Antarctic or Greenland Ice Sheets or permafrost melting. They may reduce or undo remaining margins to reach set targets. Most would add to global warming. Delays would also increase the need to rely on CO2 removal (carbon dioxide removal, CDR). There is uncertainty about the scalability of removal technologies needed to reach net-negative emissions. For example, a major avenue of CDR is the sustainable use of bioenergy (which may be CO2 neutral) combined with carbon capture and storage (BECCS). It is not fully understood how land use and land management choices for large-scale BECCS will affect ecosystem services and sustainable development (IPCC, 2018[4]). Extreme climate events, such as large-scale wildfires, can also put afforestation as well as bioenergy use combined with carbon capture and storage – key CO2 removal options – at risk. Tipping points can accelerate climate change to the point that it may irreversibly pose severe risks for health, economies, political stability (especially for the most climate-vulnerable) and, ultimately, the habitability of the planet for humans. Social and technological trends and decisions occurring over the next decade or two could significantly influence the trajectory of the Earth System for tens to hundreds of thousands of years and potentially lead to conditions that would be inhospitable to current human societies and many other contemporary species (Steffen et al., 2018[7]). Solar radiation modification could reduce some of the risks related to rising temperature but faces large uncertainties and knowledge gaps as well as substantial risks and institutional and social constraints to deployment related to governance, ethical concerns and impacts on sustainable development (IPCC, 2018[4]).
Figure 3.1. Climate change is a threat to the foundations of human well-being

Note: This chart illustrates some of the impacts of climate change that relate to land use. For example, on the food supply instabilities, the chart shows that the level of impact and risk of food supply instabilities goes up from high (red area) to very high (purple area) at global warming of around 2°C with medium confidence. Very high impact and risk means, more concretely, sustained food supply disruptions globally. For wildfire damage, the red colour means, for example, a 50% increase in area burnt in the Mediterranean region and purple, 100 million people or more affected.
Source: IPCC (2019[8]), Climate Change and Land - Summary for Policy Makers, https://www.ipcc.ch/site/assets/uploads/2019/08/4.-SPM_Approved_Microsite_FINAL.pdf.
To limit the global temperature rise to 1.5°C by 2100 with a probability of at least 50%, CO2 emissions would need to be brought to net-zero worldwide around 2050, while other sources of human-made global warming, including from other GHG emissions, would need to be at least stabilised (IPCC, 2018[4]). This is equivalent to reducing emissions by almost 9% per year. Unlike CO2, methane is short-lived so positive emissions can be consistent with constant global temperature. Objectives to reach net-zero domestic GHG emissions by 2050 hence go somewhat further than net-zero CO2 emissions targets. Countries should take into account common but differentiated responsibilities and respective capabilities as set out in the Paris Agreement. This may justify more ambitious targets for high-income countries. A target of net-zero GHG emissions in 2050 will imply net-negative CO2 emissions by 2050. Regions that mostly emit CO2, including cities, will need to reach net-zero emissions before 2050 and likely before 2045. In any case, to halt global warming on longer time scales, CO2 emissions need to become net-negative worldwide.
Box 3.1. Lessons from the COVID-19 crisis in a regional, urban and rural context
The COVID-19 crisis has revealed the close relationship between risks to the foundations of human well-being, environmental impacts and cascading systemic impacts on the economy and society. Human health risks are key. Climate change also poses risks to the foundations of human well-being, including health, with potential systemic knock-on effects. These are even bigger and on a longer time scale. They also vary across regions and cities and, as in the COVID-19 crisis, participation of local and regional decision-makers and multi-level governance has proven essential.
The COVID-19 crisis shows the importance of anticipation, preparedness and early action to mitigate human well-being and systemic risks and take cost-minimising action. They are also key to prevent and limit the well-being risks from climate change and drive down emissions while extending economic prosperity. They require integrating scientific advice in the decision-making progress among citizens, parliaments and governments at all levels.
Moving from an economic growth paradigm to well-being and sustainable development paradigm can help identify systemic risks by putting human health impacts first and thereby reinforce resilience.
The COVID-19 crisis shows that inclusiveness is key to resilience. Access of all households to adequate housing, social safety nets, including health services, water and sanitation, energy supply, adequate income, communication and education improve the resilience of societies. They will also be key in the transition to net-zero GHG emission economies and to adapt to climate change. The cost of the crisis response and the zero-emission transition will also need to be shared fairly across households and firms.
The COVID-19 crisis has shown that societies can embrace strong action to mitigate risks to the foundations of well-being but also that they are sensitive to economic risks to their livelihoods. This further reinforces the case for anticipatory, preventive action.
The COVID-19-related lockdowns have had near-term benefits for the environment, including on CO2 emissions, but at the expense of a major decline in economic activity. This illustrates how closely intertwined fossil fuels remain with economic activity. So far, GHG emissions and other environmental impacts have risen with economic activity. This link must be broken.
Small- and medium-sized enterprises (SMEs) have been vulnerable to the COVID-19 related impacts and their vulnerability has increased risks of precariousness and poverty. But they have also contributed to resilience with innovation and flexibility. Looking ahead, they may be particularly vulnerable in the zero-emission transition, as they may be more emission-intensive and find integrating new technologies more difficult. They may also have less scope to diversify activity (including geographically) as well as more limited access to financial markets and the scale economies of new technologies. Framework conditions need to put them in a position to develop business models consistent with net-zero emission economies.
It is key to identify factors that create resistance to early action. The COVID-19 crisis illustrates the governance needs to integrate governments and parliaments at all levels, the public, employers, workers and scientific advice. Vested economic interests around fossil fuels have been a major source of resistance against climate policy. Clear, democratic, participative governance structures help to identify risks and actions to mitigate them.
Globalisation has an ambivalent relationship with resilience in the COVID-19 crisis and the climate challenge. The flow of goods, services, workers and capital can alleviate asymmetric impacts across regions to the epidemic or climate change, for example, to ensure regional food supply. However, it can put local resilience at risk when global supply chains are disrupted and make local initiatives to internalise environmental costs of production more difficult.
Policies to overcome the economic impact of COVID-19 must therefore be chosen so they help advance with the just transition to net-zero GHG economies. This includes public investment and requires a place-based approach. There is a risk that stimulus packages are employed to expand well-established economic activities, especially in regions highly invested in fossil fuels and hit by the COVID-19 crisis.
Recent emission trends and emissions projected on the back of current policies are a long way off meeting these objectives. There is no sign of GHG emissions peaking in the next few years. The COVID-19 crisis has temporarily reduced emissions but with little long-term effect as emissions have fallen little with economic activity and bounce back unless energy use, land use and urban development are transformed (UNEP, 2020[9]). Policies laid out in countries’ nationally determined contributions (NDCs) to the Paris Agreement imply emissions will continue to rise (UNEP, 2019[10]). By 2030, emissions may be 27% and 38% higher than is needed to limit warming to 2°C and 1.5°C respectively. NDC commitments and current policies may be consistent with 3-degree warming by 2100 and rising beyond. Moreover, some countries are not on track to meet their own NDC commitments (Climate Analytics/New Climate Institute, n.d.[3]).
Transformations unprecedented in scale and scope are needed
To achieve these objectives, deep transformations of economic systems of unprecedented breadth over a short period of time are needed (IPCC, 2018[4]). To achieve net-zero emissions by 2050, immediate, unprecedented and ambitious actions are required (IPCC, 2018[11]; Fragkos, 2020[12]). Policy efforts often tend to focus on single and often technological solutions, without taking into account the broader infrastructural and societal implications (Chapman, 2019[13]). The close historic relationship of GHG emissions, energy use and gross domestic product (GDP) illustrates the scale of needed transformations (Figures 3.2 and 3.3).
For GHG emissions to reach net-zero, they must be decoupled from GDP in absolute terms. As the recent OECD report Managing Environmental and Energy Transitions for Regions and Cities (OECD, 2020[14]), has highlighted, relative decoupling (GHG emissions rising less than GDP) is frequent. However, examples of absolute long-term decoupling of environmental pressures are rare but absolute decoupling is needed for environmental sustainability with respect to key environmental challenges such as biodiversity loss and GHG emissions. Recently, some high-income countries have decoupled GDP from production and, to a lesser extent, consumption-based CO2 emissions (Haberl et al., 2020[15]). CO2 emissions have slightly decoupled from energy use and GDP in absolute terms since 2000 (Figure 3.3) but energy-related emissions still contribute to around 80% of GHG emissions in OECD countries, with widespread sectoral contributions to emissions across regions (Chapter 2). Some key well-being gains, such as better air quality, are negatively related to emissions.
Figure 3.2. World CO2 emissions have decoupled from GDP only in relative terms, and not from energy consumption

Note: Indices 100 in 1990.
Source: OECD (n.d.[16]), Green Growth (database), OECD, Paris; IEA (n.d.[17]), World Energy Balances, International Energy Agency.
Figure 3.3. OECD CO2 emissions may have decoupled from GDP and energy consumption in absolute terms

Note: Indices, 100 in 1990.
Source: OECD (n.d.[16]), Green Growth (database), OECD, Paris; IEA (n.d.[17]), World Energy Balances, International Energy Agency.
The three pillars of climate mitigation action – energy, land use and urban policy (New Climate Economy, 2019[18]) -- are at the heart of regional development.
Moving to net-zero emissions requires most final energy demand to be electrified and most electricity generation moved to zero-carbon sources, mostly renewable. To limit the costs and environmental impacts, energy efficiency is key. As pointed out in by the OECD and the European Union (EU) (2020[14]), the share of renewables needs to rise from 15% of the primary energy supply in 2015 to two-thirds by 2050 (IEA, 2019[19]). The energy intensity of GDP may need to fall by about two-thirds by 2050 (IRENA, 2020[20]). Technologies not yet deployed to scale, including hydrogen and carbon capture and storage, will also play an important role (IEA, 2020[21]). Urban, regional and rural policymakers will need to integrate transformations in the energy system, including shifting spatial distribution of electricity supply and energy transformation as well as stronger differentiation of pricing of electricity across time and space. They will also need to manage the activities that may be inconsistent with zero emission goals, while protecting vulnerable groups from employment loss.
Around 23% of global GHG emissions are related to land use (IPCC, 2019[22]). Food systems are responsible for one-third of global GHG emissions (OECD, 2019[1]). These include agricultural emissions as well as emissions from land use change, such as deforestation or loss of peatland. Land use is also central to net-negative CO2 emissions.
Cities account for about 70% of demand-based energy-related CO2 emissions, as the recent OECD report Managing Environmental and Energy Transitions for Regions and Cities (OECD, 2020[14]) has highlighted, following International Energy Agency (IEA) estimates based on United Nations (UN) urban population statistics. Urbanisation is expected to continue increasing, especially in middle-income countries, and is strongly associated with increases in energy demand. New buildings already need to be constructed consistent with net-zero emissions in high-income countries and all existing buildings refurbished. The complex socio-economic and geographic systems of cities and regions within which they are integrated, requires a specific approach to urban planning and energy-using activities, including transport. How related long-lived infrastructure is laid out is key. In regions where urbanisation is advancing, incorporating zero-emission development provides opportunities to provide services of cities at lower cost, while providing the mitigation, adaptation and associated co-benefits.
Subnational governments play a key role in climate change mitigation
Energy and land use relate closely to local endowments in natural resources and infrastructure which contribute to define the spatial distribution of economic activity. Local conditions are critical for defining net-zero emission strategies, for example for connecting people to jobs, for the specific industrial mix and enterprise fabric of cities and regions. Fifty percent to 80% of adaptation and mitigation actions require regional and local implementation. They have been undervalued, though in federal countries they tend to be better acknowledged (OECD, 2020[14]). Yet subnational governments have key relevant competencies for climate policy (Box 3.2).
Effective place-based action requires co‑ordinating national and international policies, to meet national and international commitments and policy frameworks. Local governments may have direct power over less than a third of urban GHG emissions reduction potential, with over two-thirds depending on either national and state governments or co-ordination across levels of government. For example, pricing of GHG emissions is cost-minimising if broad and even. Therefore, prices are ideally set at the supranational or, failing that, national level. However, CO2 emissions are priced well below international climate cost benchmarks and prices are uneven across sectors and fuels (OECD, 2018[23]). Pricing of non-CO2 emissions, such as methane emissions in agriculture, is rare. The difficulties in achieving broad pricing often reflect distributional issues. Using place-based criteria for the distribution of carbon tax revenues, as has been done in Canada, may help achieve progress. In any case, pricing is not enough. Excessively short time horizons in investment decisions and knowledge externalities call for policy instruments to guide investment, often at the regional level. There is a need to make substitutes available for high-emission activity, requiring place-based collective decisions about networks such as energy or transport.
Box 3.2. The key competencies of subnational governments in climate policy
The recent OECD report An integrated approach to the Paris Climate Agreement (Matsumoto et al., 2019[24]) has highlighted that local and regional governments have jurisdiction over crucial sectors for climate action, including buildings and parts of transportation, other local infrastructure and waste management. Many decisions taken by local authorities, such as local regulation on transport, building construction mandates, spatial planning and economic policies, determine GHG emissions directly or indirectly.
Cities and regions can also set examples of progressive emission reduction. They can develop, diffuse and implement technological and social innovations, from e-scooters to zero-carbon local housing strategies. They may be able to take action more rapidly as they have close contact with citizens and businesses, as well as strong knowledge of local conditions and capabilities.
Local authorities are well-positioned to implement national emission reduction policies and are instrumental in embedding climate action into spatial planning, infrastructure, local policies and budget through locally-tailored climate strategies in line with national objectives. Local governments play an essential role in supporting the most vulnerable as they understand the local issues faced by their citizens.
Regions, facilitate co‑ordination between the national and local levels, as well as co‑operation among local authorities. Regions have a role in climate mitigation and adaptation, given their responsibilities in several areas having an impact on economic development (Matsumoto et al., 2019[24]).
Local well-being gains can offset the cost of a net-zero-emission transition
The sharp drop in the cost of renewable electricity has lowered the cost of net-zero-emission transitions. The performance and cost of batteries, which help to integrate electricity from intermittent renewables in energy use, has also improved sharply. They are often already cheaper than fossil fuel-incumbent technologies, even without carbon pricing. Recent estimates for the UK suggest the resource cost of the zero-emission transition may amount up to 1%-2% of GDP until 2050 (Climate Change Committee, 2019[2]). Costs are concentrated on the last 10%-20% of emissions abatement. The resource cost refers to the net resources that need to be devoted to the transition, include higher investment. Negative GDP impacts of the transition are more likely and more marked in fossil fuel-exporting regions (EC, 2018[25]; OECD, 2017[26]). Negative impacts are also more likely in regions with emission-intensive activities which are difficult to decarbonise. The impact on the competitiveness of sectors subject to international competition may depend on who bears resource costs in these sectors, especially if climate policies proceed at unequal speed across countries. For example, if taxpayers assume resource costs in such sectors, competitiveness in these sectors may be preserved, and resources would not need to be reallocated to other sectors. Such reallocation may or may not affect national GDP (OECD, 2017[26]) but would further impact the distribution of economic activity across regions.
Investment in research and development (R&D) and deployment of zero-emission technologies can lead to more rapid cost reductions than projected and such cost reductions could also boost economic activity (New Climate Economy, 2019[18]). Regions that attract such investment may benefit the most. For example, the cost of producing and using hydrogen – which can be produced with zero emissions and allows long-term storage of energy – has fallen substantially since 2019. Such cost declines may be the most likely in replicable, modular technologies, such as in renewables and batteries. They have not occurred in nuclear energy nor, so far, in carbon capture and storage (Climate Change Committee, 2019[2]). Policies to boost zero-emission-consistent investment and innovation bring about such cost reductions. The GDP impact of decisive climate action may also turn positive if accompanied by GDP-boosting structural reforms and zero-carbon consistent investment, while carbon tax revenues could deliver substantial public debt reductions (OECD, 2017[26]).
Achieving net-zero GHG emissions can result in substantial well-being gains, which can offset these costs including human health. These benefits range from health and productivity to reducing energy poverty. Overall, the societal well-being gains of climate neutrality remain largely unaccounted for (IPCC, 2018[4]). A recent quantified assessment for the UK includes time gains from less traffic congestion as well as health improvements from a shift to active mobility (walking, cycling), from less meat-intensive diets, improved air quality and reduced traffic noise (Climate Change Committee, 2019[2]). These estimates suggest that welfare impacts could reach a similar magnitude as the costs in high-income, fossil-fuel importing countries and regions. The estimates are a broad approximation and do not include well-being benefits which are difficult to quantify in monetary equivalents, including: health benefits from improved housing quality; lower water and air pollution; improved biodiversity; climate resilience and recreational benefits from transformations in land use and agriculture. The benefits of reduced air pollution may also be underestimated given the increased evidence of the damaging impact of air pollution on a broad range of human well-being and health outcomes in recent years. Economic outcomes may also improve with health. Lower air pollution (Dechezleprêtre, 2019[27]) also boosts productivity. Several studies find that air quality co-benefits offset a large proportion of climate policy costs (Karlsson, Alfredsson and Westling, 2020[28]). For the East Asia region, the co-benefits of climate change mitigation in terms of human health may reach 6% of GDP, when also including the impact on adaptation costs. This exceeds the estimated cost of mitigation of 2% of GDP (Xie et al., 2018[29]). Focusing on urban contexts, expenditures may be mostly offset by the local co-benefits. Welfare effects may be strongly positive yet with slightly negative GDP and employment effects. Economic co-benefits of climate change mitigation policies in urban mobility, for example, can be put forward as a forceful argument for policymakers to take action (Wolkinger et al., 2018[30]).
Well-being gains associated with emission reductions need to be included in project appraisal. To scale up and deploy finance for environmental and energy transitions, well-being gains should be integrated in cost-benefit analysis. Environmental and social criteria can be included in cost-benefit analysis to make environmental costs and benefits part of a broad economic analysis (OECD, 2020[14]). For example, the valuation of biodiversity and ecosystem services helps monetise environmental impacts of policies and investment projects (OECD, 2018[31]). Appraisal guidance may need to be updated to reflect these benefits.
Despite potentially low overall costs overall, within countries, mitigation measures may have more profound impacts on some regions than others, and households and businesses in these regions will need to be actively supported through the transition to avoid creating new geographies of discontent and societal pressures that may, in turn, slow progress towards the zero-carbon goals. Central and supranational regional policy to address differences in regional impacts on well-being, employment and GDP and identify vulnerable regions and individuals and compensate them. Policymakers will also need to pay attention not to reward investment that has been inconsistent with the zero-emission transition, as this would harm incentives for appropriate investment going forward.
Early action is key to avoid high costs
Good policy design is vital to keeping costs low and maximising benefits including a stable long-term direction with clear governance, regular reviews for flexibility, use of markets to find the best solutions, support for large-scale deployment and R&D of new technologies (Climate Change Committee, 2019[2]). Integrating climate policy into development plans promotes synergies to reduces climate change risk and enhance resilience, while also helping to minimise costs. Regional, urban and rural policies will be discussed in more detail in the following chapter.
Delayed action raises costs globally but also locally, in cities and regions where the delays occur. The costs of delaying action to stabilise GHG for a 1.5℃ may be USD 5 trillion per year (7% of annual world GDP) (Sanderson and O’Neill, 2020[32]). Higher local costs result because later reductions will require faster expansion of new technologies, raising susceptibility to errors (Chapman, 2019[13]). Moreover, if investment in long-lived capital goods and infrastructure is not consistent with the zero-carbon transition, it would need to be written off before its economically useful life, resulting in wasted investment spending. Stopping investment in infrastructure that is inconsistent with the net-zero-emission transition is key to avoiding unnecessary costs. Current and stated policies imply investment paths that are inconsistent with reaching the Paris objectives, resulting in increasing stranded asset risks over time. Stranded asset risks are particularly large in fossil fuel supply (Figure 3.4).
Figure 3.4. Energy investment with current or stated policies differs sharply from investment needed to meet the Paris Climate Agreement

Note: The Current Policies Scenario shows what happens if the world continues along its present path, without any additional changes in policy. The Stated Policies Scenario incorporates today’s policy intentions and targets. The Sustainable Development Scenario maps out a way to meet sustainable energy goals, including limiting global warming to well below 2°C and lowering air pollution while providing universal access to energy.
Source: OECD-EU (2020); IEA (2019[33]), World Energy Outlook 2019, https://www.iea.org/reports/world-energy-outlook-2019 (accessed on 3 April 2020).
Regions heavily invested in fossil fuel extraction and transformation are therefore particularly at risk from stranded assets. This applies in particular to those regions where extraction and processing costs are particularly high. Production in these regions is priced out of the market first when fuel prices fall. Stranded asset risks are also higher if a large share of this cost is undertaken upfront when extraction projects are undertaken. For example, a decisive transition to net-zero emissions in major oil-importing economies would result in substantial GDP losses in oil-producing regions in Canada and the United States (US), where oil is extracted at higher costs than by other supplying regions (Mercure et al., 2018[34]).
Stranded assets concern all regions and cities. For example, new buildings need to be zero-emission-consistent today to avoid needing to be refurbished at a higher cost later. Since cars are used for about 15 years in high-income countries, purchases of new cars with internal combustions engines in countries and after 2035 would be sub-optimal in countries and regions with net-zero CO2 emission targets for 2050. The projected availability of low-cost electric cars suggests that the sale of new cars with internal combustion engines should be phased out even by 2030 (Climate Change Committee, 2019[2]) as announced in a few countries, such as the UK. Delayed action also means forgoing the local well-being and environmental benefits of emission reduction.
Transitions need to be inclusive within and across regions
Only a just transition can garner broad engagement and support. Climate change threatens the livelihoods of poor and vulnerable households, firms and regions the most. As the COVID-19 crisis has illustrated, broad support among citizens and the business community for policies to mitigate makes it easier to respond to systemic challenges. The well-being benefits of the zero-carbon transition can benefit vulnerable households that, within metropolitan areas, often live in areas most exposed to air and noise pollution. At the same time, job loss and adapting to zero-carbon modes of moving or housing and related costs are particularly difficult for low-income households that have fewer opportunities and resources to adapt.
A just transition approach involves an explicit focus on how a policy could be used to benefit disadvantaged groups and to take active measures to address economic inequalities and mitigate regressive outcomes as argued in Managing Environmental and Energy Transitions for Regions and Cities (OECD, 2020[14]). The pathway to positive equality outcomes involves carefully considering who might be impacted by a given policy and involving these groups or communities in the decision-making process (Chapman, 2019[13]). Policy measures with potentially negative impacts on household income or livelihoods must be accompanied by corresponding mitigating measures, such as exemptions, subsidies, compensation for losses and concrete support to help affected individuals and communities. In policy implementation, utilising the local workforce where possible can help achieve an equitable distribution of benefits at the local level, or by training local unemployed people to fill new jobs, and by ensuring that new employment opportunities do not exacerbate existing inequalities (Ürge-Vorsatz, Boza-Kiss and Chatterjee, 2019[35]). A wider public needs to be aware of the required transition and needs to be involved in future benefits (Chapman, 2019[13]).
Adaptation will have to complement mitigation but it cannot be a substitute. Global warming of 2°C and higher poses risks for human well-being which adaptation may not be able to counter. For example, cities can cope with a 20-30 cm rise in sea level by building dams and other forms of protection. However, if the sea level rises by several metres, such a dam no longer helps.
Place-based adaptation is needed as a complement of decisive mitigation
Warming of up to 1.5℃ is inevitable and humanity must adapt to it. Article 7 of the Paris Agreement recognises adaptation as a global goal and key to protect human livelihoods and ecosystems. Humans have always adapted to changing environmental conditions but anthropogenic climate change from GHG emissions poses a particular challenge as the speed of change is unprecedented. Moreover, today’s larger population implies an increasing number of people at climate risk, especially vulnerable low-income households in highly exposed regions with weak physical social and knowledge infrastructure. The current interconnected globalised economy makes it more vulnerable through supply chain disruption, resulting in differentiated place-based impacts (Okazumi and Nakasu, 2015[36]).
Extreme weather events are increasingly related to climate change. Climate-related hazardous events show an increasing trend during the period 1980 to 2016 for economic loss and human lives (Formetta and Feyen, 2019[37]). Flood- and wind-related events dominate reported events and wind caused 40% of fatalities from extreme weather events. However, economic losses may be underestimated as they are usually estimated using disaster databases that report only insured losses and do not cover indirect and intangible damages (Forzieri et al., 2018[38]).
Figure 3.5. Hazards and their impacts from 1980 to 2016

Source: Formetta, G. and L. Feyen (2019[37]), “Empirical evidence of declining global vulnerability to climate-related hazards”, https://doi.org/10.1016/j.gloenvcha.2019.05.004; with data from Munich Re (n.d.[39]), NatCatServices (database), which reports insured and direct losses only.
Without adaptation, consequences are substantial. For instance, with current climatic conditions, 1 in 5 people in cities are exposed to flood and 6% of cities risk being entirely flooded, as a recent OECD CFE report, Cities in the World: A New Perspective on Urbanisation, has shown (2020[40]). Worldwide flood loss from sea level rise of 40 cm in 2050 in 136 coastal cities is projected to reach USD 1 trillion per year compared to USD 6 billion in 2005 (Hallegatte et al., 2013[41]). Around 63 million people per year would be at risk of flooding even under a 1.5℃ warming (IPCC, 2018[11]). Exposure to a higher temperature and heatwaves raises mortality substantially, even under 1.5°C (Zhang et al., 2018[42]). Without adaptation, damage from multiple climate hazards to the present stock of infrastructure, for example in Europe, is projected to increase more than tenfold, from EUR 3.4 billion/year in 2010 to EUR 37 billion/year by 2100 (Forzieri et al., 2018[38]). The estimated damage could be much larger if interdependent and cascading damages are included. The additional impact of a one decimal increase in temperature is higher, the higher the temperature.
Adaptation can reduce climate risk substantially and more effectively under 1.5℃ warming than under 2℃ warming (IPCC, 2018[11]) (Box 3.3). Adaptation can also generate additional benefits, including leisure benefits of nature and environmental awareness (Kim and Song, 2019[43]). It can also stimulate innovation, which in turn can boost economic activity (Global Commission on Adaptation, 2019[44]). Green Infrastructure (GI) is especially notable for multiple benefits alongside adaptation (Box 3.4).
Box 3.3. Examples of quantified adaptation benefits
Taking decisive adaptation action such as reducing coastal flood risks in 136 cities in the face of socio‑economic vulnerability can reduce flood loss from USD 1 trillion to USD 52 billion by 2050 (Hallegatte et al., 2013[41]). Decisive adaptation action in Alaska was found to reduce expenditure on infrastructure by USD 2.3 billion this century (Melvin et al., 2017[45]). Climate-proofing existing infrastructures generate a benefit-cost ratio of four, only counting avoided losses from future climate hazards. Better drainage and flood barriers can generate positive returns for 60% of the roads that are exposed to at least a single flood event by investing just 2% of road value (Koks et al., 2019[46]). Investing USD 1.8 trillion could generate a net benefit of USD 7.1 trillion by 2030 (Global Commission on Adaptation, 2019[44]).
Climate change can exacerbate pre-existing social inequalities and social conflict (Hsiang et al., 2017[47]; Hsiang and Burke, 2014[48]). The marginalised and the poor bear the highest costs of damage relative to their income and are disproportionately at higher risk to hazards (Winsemius et al., 2018[49]). Elderly people, women and those with lower education level are more vulnerable to increased temperature variation for example (Marí-Dell’Olmo et al., 2019[50]). Part of the poverty-increasing impact of climate change comes from the adaptation of markets. For example, climate change will induce changes in land prices which will make poor people live and work where they are more exposed. Food-importing regions will be more affected by rising food prices and poor populations will be the most affected. Indigenous peoples too are especially vulnerable to the impacts of climate change since they usually live in vulnerable environments, including small islands, high-altitude zones, desert margins and the circumpolar Arctic (Nakashima et al., 2012[51]). For instance, in Canada’s Northern regions, this is having increasingly widespread repercussions on the life of Northern Peoples, such as with respect to food, their environment and ecology (OECD, 2020[52]). Regional governments will need to counter these trends, bearing in mind that rising poverty may further reduce the poor’s representation in adaptation decisions. They will also need to emphasise preventive action to avoid moral hazard.
Hence, inclusive regional development not only reduces poverty and improves resilience to a broad range of socio-economic shocks but also resilience to climate change. Improving inclusive outcomes in terms of regional convergence of productivity, access to basic infrastructure services, notably health, water and sanitation and modern energy by 2030, as in the UN Sustainable Development Goal (SDG) objectives, as well as good social income protection systems diminish the impact of climate change on poverty substantially. Strong mitigation action will prevent the poverty-increasing effects of climate change.
Box 3.4. Benefits of Green Infrastructure (GI)
Flood mitigation works that include GI components can provide multiple benefits, including energy saving due to the thermal insulation provided by green roofs as well as carbon sequestration, surface temperature reduction provided by pervious pavement and emissions reduction from energy saving (Alves et al., 2019[53]). The benefits net of cost were valued at EUR 2.91/m2/year for the Sint Maarten Island in the Netherlands (Alves et al., 2019[53]).
The multifunctionality of GI was demonstrated in a case study of 447 projects reporting 964 benefits (Kim and Song, 2019[43]), which in broader terms were socio-cultural benefits, potential reduction in grey infrastructure needs, flood protection and ecosystem protection among others. For example, a 20% increase in green space can provide heat island reduction benefits even in cities with cool temperate climates, reducing local surface temperature peaks by 2℃ (Emmanuel and Loconsole, 2015[54]). This can reduce energy demand for cooling and thereby also contribute to mitigation. Urban green space also enhances residents’ well-being in its own right (Hiscock et al., 2017[55]).
Limitations of GI mostly arises from under-researched and therefore unclear outcomes (Sussams, Sheate and Eales, 2015[56]). For example, there are few studies to determine the full range of health and other well-being outcomes (Venkataramanan et al., 2019[57]). Incorporating these well-being outcomes can significantly increase perceived co-benefits, boosting adaptation (EC, 2019[58]).
Regional governments need to embrace adaptation beyond built infrastructure and disaster management
Adaptation costs and benefits arise mostly locally (Greenhill, Dolšak and Prakash, 2018[59]) but benefits also cross boundaries. For example, in 2011, floods in Thailand affected the global information technology (IT) and automobile industries through ruptures in supply chains in Japan in particular (Okazumi and Nakasu, 2015[36]). Multi-level governance is key, including partnership among civil societies, businesses and communities (IPCC, 2012[60]; 2018[11]). The need to strengthen resilience through greater co-ordinated effort was also demonstrated during the COVID-19 health pandemic (OECD, 2020[61]).
The simplest description of adaptation is building resilience to climate risk with disaster management (Dolšak and Prakash, 2018[62]; IPCC, 2018[11]) and preparedness of physical infrastructures. Physical infrastructure includes nature-based “green” and aquatic-based “blue” infrastructures, in addition to the traditional grey structures (Alves et al., 2019[53]). But adaptation is also underpinned by socio-cultural and political systems (Grecksch and Klöck, 2020[63]; Pelling, 2010[64]) leading to different levels of exposure and vulnerability (IPCC, 2014[65]; 2012[60]). Focusing only on physical infrastructure and tangible assets loses track of impacts on social well-being especially among on marginalised and poor people who bear the highest costs of damage relative to their income and are disproportionately at higher risk to hazards (Winsemius et al., 2018[49]). These different dimensions can be integrated into a broader definition of infrastructure, to encompass physical, social and knowledge infrastructures (Figure 3.6).
Figure 3.6. The three integrated infrastructures of climate change adaptation (CCA)

An explicit focus on social infrastructure facilitates an inclusive approach. Social infrastructure includes people, their networks and culture. Social networks at the local level can identify vulnerable people and exposed physical infrastructure. They also create sources of resilience through mutual support, such as through business organisations or neighbourhood associations. Subnational governments are well-placed to draw on the resources of local networks. In rural regions in particular, regional governments are needed to co‑ordinate local government action, as rural municipalities are often very small. Community views on climate impacts on their livelihoods can help uncover and overcome exposure and vulnerability and increase communities’ willingness to participate in adaptation (Krauß and Bremer, 2020[66]).
Knowledge infrastructure (Taylor et al., 2020[67]) includes both formal and informal knowledge and its integration in decision-making (Box 3.5). For example, experiential local knowledge and context-based rich narratives can be added to existing geographical information tools (Taylor et al., 2020[67]). They can drive policy discussion towards alternative perspectives on building urban resilience. Integrating these features in adaptation requires transforming the governance system. (Flores et al., 2019[68]). For example, the European Climate Adaptation Platform (Climate-ADAPT), a partnership between the European Commission (EC) and the European Environment Agency, was created to learn and share adaptation experiences. Drawing on formal and informal knowledge may help improve the range of costs and benefits taken into account in project appraisal, especially in the context of unknown climate events and systemic risks.
Box 3.5. Knowledge infrastructure
Formal knowledge is scientific or technical. It includes any technology used for data and information flow such as early warning and observatory systems, which are primarily used for preventive adaptation. Informal knowledge consists of local practices and Indigenous experience (Krauß and Bremer, 2020[66]), which can identify local context-specific vulnerabilities and impacts. It requires stakeholder engagement and a participatory approach. Integrating local knowledge alongside formal scientific knowledge enriches the knowledge base (Ainsworth et al., 2020[69]) in the sense of filling the gaps that may exist within each of these knowledge domains. While policy decisions need to be based on scientific knowledge, decisions to make the most of synergies and trade-offs require local and informal knowledge, as the COVID-19 crisis has illustrated. This also improves the chances of collective support.
Climate change will pose challenges to both urban and rural areas (OECD, 2020[14]). Local urban heat islands, for instance, can increase local temperatures and modify meteorology in cities. These effects can damage physical and social infrastructure, and increase energy demand for space cooling, further driving up energy demand during higher peak loads. Tree cover has major impacts on land surface temperature in heatwaves. Much urban population lives in low-lying coastal areas.
Rural areas may face future increased food market volatility, shifts and losses in plant and animal species and, depending on the region, increased water scarcity, coastal erosion or wildfires. Changes in the timing of seasons, temperatures and precipitation will also shift the locations where rural land-based economic activities, like agriculture, forestry and tourism can thrive. More workers in rural areas may work outdoors, exposing them more strongly to weather-related risks, such as excessive heat. Both urban and rural areas are expected to experience major impacts on water quality and quantity, resulting in fierce competition between water uses. Consequently, water policies need to be adjusted to changing local conditions and water governance frameworks to manage trade-offs across water users, rural and urban areas, as well as generations will need to be implemented (OECD, 2021[70]).
City and regional government agencies and organisations have developed adaptation plans and policies. Examples include disaster risk management, infrastructure systems, agricultural adaptation and public health. Adaptation requires co‑operative private sector and governmental activities and integration with a broad range of policies, for instance on land use planning, resource management and health. Current efforts are insufficient. Most businesses do not get involved in adaptation for example. In the UK, only 20% take preventive action, by following up on risk assessments periodically and by identifying and implementing solutions (GRI, 2019[71]). This is unlikely to be cost-effective. In high-income OECD countries, much progress has been made in identifying local hazards and making this information available. However, this is less true for regions in low-income countries. As a result, adaptation spending is skewed and does not correspond to vulnerabilities.
Higher resolution climate modelling is needed to identify regional climate change hazards and appropriate adaptation action. More precise regional attribution of drought and flood risks for example will allow better-targeted adaptation action. This may require centralising climate modelling research at the continental level to bundle the resources needed for high-resolution modelling of climate impacts. Close co‑ordination of climate modelling with regional and local policymakers, who understand local vulnerabilities, will also enable climate modelling to respond to local development needs. Integrating climate modelling and regional development is therefore important (Shepherd and Sobel, 2020[72]).
Systemic risks from a simultaneous breakdown
Regions are increasingly physically interconnected through trade and digital information flows. This makes them more vulnerable to system failures and breakdown where a single climate hazard can lead to cascading extreme events (Pescaroli et al., 2018[73]) also referred to as systemic risks (OECD, 2003[74]). It can occur with “unusual combinations of processes” (Zscheischler et al., 2018[75]). Climate change may well add to such systemic risks as they are without historical precedence. It can complicate understanding, preparation and prediction of future hazards, which interact with socio-economic transformations, such as automation (Colvin et al., 2020[76]). Cascading risks are growing with climate risk for systems such as critical infrastructures (Suo, Zhang and Sun, 2019[77]). They may be non-linear, stochastic and interconnected (Renn, 2016[78]). Box 3.6 provides examples.
Box 3.6. Examples of cascading events
The 2011 Chao Phraya floods in Thailand, where 744 people lost lives and economic growth decreased by 3.7% alongside chain-reaction damage across the globe through supply chain disruptions for the automotive and IT industries.
The 2011 tsunami in Japan destabilised the Fukushima nuclear plant with a loss of 15 891 human lives and a damage cost of USD 140 billion (Okazumi and Nakasu, 2015[36]) with supply chain disruptions in electronics components.
The 2017 flooding in Houston, US, caused by Hurricane Harvey, resulting in the explosion and fire of a chemicals plant. The US is inflicted with such disaster on a yearly basis, costing between USD 6 billion to 16 billion (Cutter, 2018[79]).
The cost of such cascading risks when accounting for their interactions is more than the sum of individual events (Zscheischler et al., 2018[75]). This reinforces the need for coherence between disaster risk reduction (DRR) and climate change adaptation (CCA) (OECD, 2020[80]).
The ongoing COVID19 health pandemic reminds us that regions need to be resilient to socio-economic risks, which are the consequence of uncertain cascading events. It further shows that cascading events can undermine socio-economic development more generally. For example, the cascading impacts of COVID 19 caused supply chain disruption and lowered investment confidence across the globe, as well as the human development index to turn negative (UNDP, 2020[81]; OECD, 2020[61]).
To tackle systemic risks (Dodman et al., 2019[82]), top-down impact modelling should be complemented with science-based storytelling, to allow societies to understand the implications, and bottom-up approaches, allowing local and regional key stakeholders to engage collaboratively (Zscheischler et al., 2018[75]). This requires the need for integration of different knowledge paradigms and multi-level governance. To test the readiness and adaptive capacity of critical infrastructures, low probability events with high potential impact need to be considered, including weather events (Pescaroli et al., 2018[73]).
Strengthening resilience to systemic crisis events with unknown knock-on effects requires a holistic and participatory approach (Edwards, 2009[83]; OECD, 2003[74]). An example is the “4 Es” framework: Engagement, Education, Empowerment and Encouragement (Edwards, 2009[83]). Individual citizens and communities need to engage with the climate policy agenda to gain trusted information. Critical reflection on this information empowers them to participate in decision-making and encourages them to respond quickly to problems before they fully materialise. This is vital to reduce disaster risks. Bounded rationality may however limit these responses when humans are faced with something they have never experienced. Governments, therefore, need to elaborate choice options to build resilience (Edwards, 2009[83]). This can be facilitated by local and regional councils providing dedicated government staff and going beyond communicating towards engaging with individuals and communities for better collective and individual decisions. Educating the communities on risk management should connect to their ways of living, which can empower them to act. This shows the need for place-based resilience programmes within the purview of local and regional governments.
Where do regions stand: Indicators of progress, well-being impacts and vulnerabilities
GHG emissions across OECD regions: Many variations across territories but not over time
Metropolitan regions contribute about 60% of production-based GHG emissions (excluding emissions from land use and land use change) across OECD countries (Box 3.7, Figure 3.7). However, metropolitan emissions are the lowest per capita (Figure 3.8). Remote rural regions may emit three times more per capita than large metropolitan regions, illustrating the extent of transformations of economic activities required in remote regions. The remote rural regions’ contribution to total emissions is the largest in transport and industry. They also make the largest contribution to agricultural emissions. In metropolitan regions, emissions from electricity generation as well as the residential sector also make large contributions. Regional production-based emissions are particularly useful to understand where production will require the most transformation and related policy action.
Across all region types, per capita emissions have fallen little since 2010. Emissions and GDP per capita are positively correlated and more clearly so if the highest-emitting regions are not considered (Annex Figure 3.A.1). This relationship would be clearer if emissions were measured on a demand basis rather than on a production basis, as high-income regions consume more goods and services, including emission-intensive ones. Yet some high-emitting activities, such as heavy industry are located in low-income countries with low consumption of goods and services. Indeed, among regions with high GDP, the spread in emissions per capita is particularly wide. In those high GDP regions with low per capita production-based emissions, demand-based emissions are still likely to be high.
Figure 3.7. Metropolitan regions emit the most greenhouse gas emissions

Note: OECD countries, Bulgaria and Romania. GHG emissions excluding emissions from land use and land use change.
Source: OECD calculations based on EC (2020[84]), EDGAR - Emissions Database for Global Atmospheric Research, Joint Research Centre, European Commission.
Figure 3.8. Greenhouse gas emissions per capita are highest in remote regions

Note: OECD countries, Bulgaria and Romania. GHG emissions excluding emissions from land use and land use change.
Source: OECD calculations based on EC (2020[84]), EDGAR - Emissions Database for Global Atmospheric Research, Joint Research Centre, European Commission.
Box 3.7. Regional greenhouse gas emission data
GHG emission data are mostly collected nationally and internationally. Regional data are collected using local emission inventories only in a few countries. Where available, they are typically not comparable across countries. Sectors may be defined differently and regionally collected data do not always cover all emissions. In this OECD Regional Outlook report, regional emissions are estimated on the basis of the Emissions Database for Global Atmospheric Research of the EC’s Joint Research Centre (EDGAR, JRC 2020). It attributes national GHG emissions to locations according to about 300 proxies for 26 main sectors and subsectors, depending on the type of technology and International Energy Agency (IEA) fuel types, following Intergovernmental Panel on Climate Change (IPCC) reporting formats and guidelines. Locations of emissions are identified with various sources of spatial research (Janssens-Maenhout et al., 2019[85]). The emissions data used in this study are from Crippa et al. (2020[86]) for CO2, while non-CO2 GHG emissions data are from EDGAR.1 Total GHG emissions are expressed as CO2 equivalents calculated using the 100-year global warming potential in the IPCC 5th Assessment report (AR5). The proxies capture a substantial part but not all of the local emission determinants. For example, residential emission estimates capture buildings and population but not the degree of building insulation. Location estimates of agricultural emissions capture the number and species of ruminant animals but not how they are fed.
The emissions are attributed to five sectors:
1. The power supply sector contains all combustion of fuels for electricity and heat generation.
2. The industry covers the whole value chain from mining primary materials to manufacturing and recycling products. They include energy use process emissions and fugitive emissions.
3. Agriculture includes agricultural soils, agricultural waste burning, enteric fermentation and manure management.
4. The residential sector includes buildings and waste.
5. Transport encompasses freight and passenger ground, sea and air transport.
Residential emissions are attributed spatially using high-resolution criteria on population and built-up density. The dataset classifies six categories of human settlements (mostly uninhabited rural and dispersed rural areas, villages, towns, suburbs and urban centres) using satellite imagery. These data are combined with population density from the latest population censuses. Emissions from combustion in the household and commercial sectors are attributed to total population density maps for all fossil fuels.
Agricultural emission sources are attributed spatially according to agricultural land use, soil type, local livestock density and crop type datasets and maps from the UN Food and Agricultural Organization (FAO). Fuel combustion emissions in the agricultural sector are distributed over “rural” areas (mostly uninhabited rural and dispersed rural areas) for all fuels with the exception of natural gas which is assumed to be used mainly in cities, towns, villages and to a lesser extent in rural areas. A fishing map is also used.
Industrial and power sector emissions are mainly located at the plant location co‑ordinates on point source grid maps. Power plant emissions have been distributed according to the point source distribution data sets, including intensity parameters and differentiating the fuel types coal, gas and oil. However, data on the location of power plants date back to 2012. A specific proxy captures emissions in the non-metallic minerals production (mainly cement and lime) for the world-leading producers of cement based on the plant locations and annual throughput. The difference between the total of the facility emissions and the country total of the given sector is distributed using urban population data. Gas flaring activities are distributed on night-time light data for areas with strong gas flaring activities such as the North Sea Region. The co‑ordinates of coal mines help locate related emissions, distinguishing between hard and brown coal mines.
Transport route information is used for the spatial attribution of transport emissions. Different proxy data layers for three road types worldwide (highways, primary and secondary, residential and commercial roads) are obtained from OpenStreetMap and combined with different weighting factors for the emission distribution for each road type, depending on the type of vehicles circulating on the type of roads. While the intensity of road use is not directly taken into account, the proximity of secondary roads may capture some road intensity use. Similar data are used for railways and inland waterways. For maritime traffic, identification and tracking data are used and for air, data from International Civil Aviation Organisation as well as flight information, taking into account flight patterns and their role in emissions (landing/take-off cycle).
For residual emissions which cannot be located, especially in the industrial and power sector, population-based gap-filling techniques are used.
To get a sense of the accuracy of the regional emissions estimates based on the EDGAR model from the EC JRC, the values can be compared to the regional emissions data published by the national statistical offices (NSOs) for those countries for which they are available. These include Australia, Belgium, Canada, the Netherlands, New Zealand, Sweden, the UK and the US. It is not a perfect comparison because the scope of emissions in both sources rarely match exactly (e.g. not all sectors or not all types of GHGs may be covered by the NSOs). Still, there is a high correlation between both sources in the emissions values for the same region (both for small [TL3] and large [TL2] regions). Since the emissions data published by the NSOs cover on average only 88% of emissions covered by the EC JRC, the EC JRC estimate is usually higher than the NSO value for the same region. However, in a few regions, EC JRC estimates and NSO values differ significantly. The two most extreme examples are Alberta and the Australian Capital Territory. The EC JRC estimated emissions for Alberta are only half those measured by the Canadian NSO, while the EC JRC estimate for the Australian Capital Territory is ten times those measured by the Australian NSO.
In the US, the North American Carbon Program (NACP) has followed a similar approach as the EC JRC. Their Vulcan database estimates CO2 emissions using emissions factors and spatial information on industrial and electricity generation facilities and roads among other things. For 48 US cities, the estimated CO2 emissions were compared to self-reported emission inventories of those cities (Gurney et al., 2021[87]). On average, the self-reported emissions are smaller than the estimated emissions.
Rural regions emit the most GHGs in per capita terms in most countries (Figure 3.9). Within-country regional variation in emissions is larger than between countries (Figure 3.10) and there is much variation in agriculture, power generation and industry-related emissions (Figures 3.11 and 3.12). The highest-emitting regions are in Australia, Canada, New Zealand and the US. In these regions, emissions related to power generation and industrial emissions dominate. High power and industry emissions often entail higher transport emissions, likely reflecting freight in getting fossil fuels or industrial production to final demand. Energy-supply-related activity and energy-intensive industry are capital-intensive and capital goods are often long-lived. These regions may therefore be subject to substantial stranded assets risks. Regions with the highest agricultural emissions are in Australia, Chile, New Zealand and the US. Since 2010, emissions per capita have not fallen substantially in most of them.
In some top-emitting regions, GDP per capita is particularly high, especially in North America, highlighting the entailing economic and financial risks which need to concern policymakers, especially in high-emission regions, beyond those shown here. This may also concern intensive agricultural regions, where agricultural production is capitalised in land values. These regions do not, however, stand out in terms of life satisfaction, for which differences are much smaller, perhaps because much of the GDP accrues to capital owners who do not reside in the region (Annex Figure 3.A.2).
Figure 3.9. In most countries, rural regions have the highest emissions per capita

Source: OECD calculations based on EC (2020[84]), EDGAR - Emissions Database for Global Atmospheric Research, Joint Research Centre, European Commission.
Figure 3.10. Within-country variation is larger than between countries

Source: OECD calculations based on EC (2020[84]), EDGAR - Emissions Database for Global Atmospheric Research, Joint Research Centre, European Commission.
Figure 3.11. Agricultural emissions per capita are particularly high in New Zealand

Source: OECD calculations based on EC (2020[84]), EDGAR - Emissions Database for Global Atmospheric Research, Joint Research Centre, European Commission.
Figure 3.12. Industrial emissions per capita are high in Australia, Norway and North America

Source: OECD calculations based on EC (2020[84]), EDGAR - Emissions Database for Global Atmospheric Research, Joint Research Centre, European Commission.
Figure 3.13. Energy emissions per capita are high in some Dutch, Finnish, Greek and US regions

Source: OECD calculations based on EC (2020[84]), EDGAR - Emissions Database for Global Atmospheric Research, Joint Research Centre, European Commission.
Figure 3.14. In most of the highest-emitting regions, energy supply, transport and industry-related emissions dominate

Source: OECD calculations based on EC (2020[84]), EDGAR - Emissions Database for Global Atmospheric Research, Joint Research Centre, European Commission.
Few regions have yet reached CO2 emissions which are close to zero. Many OECD regions have set a net-zero GHG emission target, which will in practice mean reaching net-negative CO2 emissions to offset positive non-CO2 emissions, notably methane. The emissions data shown here do not capture carbon sinks, as they exclude emissions from land use and land use change, which can be negative. However, afforestation and reforestation, a major potential carbon sink, can in any case only absorb a flow of CO2 emissions in the growth phase. All large regions (TL2) with estimated production-based emissions below 2.5 tons of CO2 are located in middle-income South and Central American regions (Figure 3.15) except the Swedish capital Stockholm and Romania’s North East region. These regions typically do not host CO2 intensive power supply but also have much lower transport emissions per capita.
Figure 3.15. Some OECD regions emit little CO2, mostly in middle-income regions of South America

Source: OECD calculations based on EC (2020[84]), EDGAR - Emissions Database for Global Atmospheric Research, Joint Research Centre, European Commission.
Most regions need to move more decisively to renewables in electricity generation
Moving towards a low-carbon economy is central to halting global warming. Since much energy use, for example in transport, needs to be electrified in the transition to net-zero emissions by 2050, progress in moving to zero-carbon electricity generation needs to be particularly rapid, so energy supply can move to decarbonised electricity. Electrification of end-use sectors can then contribute to decarbonisation in a cost-effective and timely way. Yet, the transition to zero-carbon electricity production remains unequal across OECD regions.
As highlighted in Regions and Cities at a Glance 2020 (OECD, 2020[88]), for the same amount of electricity production, high‑carbon-intensive regions release, on average, 23 times more tons of CO2 than low‑carbon-intensive regions within each country. Behind such stark inequalities in carbon efficiency is the shift towards renewable sources for electricity production (see next section). Rural regions are less carbon-intensive in electricity production than metropolitan regions, generating 27% of the electricity but only 20% of the CO2 (Figures 3.16 and 3.17). These differences also have implications for regional development. As the following sections show, many regions are far off near-term benchmarks for meeting the Paris Agreement.
Figure 3.16. Rural regions are less carbon-intensive in electricity production

Source: OECD (2020[88]), OECD Regions and Cities at a Glance 2020, https://doi.org/10.1787/959d5ba0-en.
Figure 3.17. Regional disparities in CO2 emissions of electricity generation can be large

Source: OECD (2020[88]), OECD Regions and Cities at a Glance 2020, https://doi.org/10.1787/959d5ba0-en.
Most OECD countries still have regions that rely on coal-fired electricity generation
As the most carbon-intensive energy source, coal will be the first fossil fuel that will have to be phased out in electricity generation. Indeed the IEA Sustainable Development Scenario (IEA SDS), which is consistent with the Paris Agreement, indicates that the average share of coal-fired electricity generation across OECD countries should be no more than 6.5% by 2025 and fall close to zero by 2040 (Figure 3.18). Coal use for electricity generation should be nearly extinguished by 2050, also in developing economies. Countries, regions and cities with net-zero-emission objectives by 2050 may need to exceed IEA SDS benchmarks. The Powering Past Coal Alliance, in which many OECD countries are members, has set a more stringent benchmark. It argues that the share of coal-fired electricity generation across the OECD should be phased out completely by 2031 for cost-effective climate mitigation consistent with the Paris Agreement (Climate Analytics, 2019[89]).
Figure 3.18. To be aligned with the goals of the Paris Agreement, coal-fired electricity should be largely eliminated by 2030
Currently, 167 out of 425 (39%) large OECD regions (TL2) are above the 2025 IEA benchmark for OECD countries. Among 37 OECD and partner countries for which data are available, only 7 countries are coal-free in all regions (Figure 3.19). Twenty-three countries still have at least 1 region where coal accounts for over 50% of electricity generation. Within countries with regions that still use some coal, it is usually concentrated in some (Figure 3.20). Countries where all regions hosted coal-fired electricity generation in 2017 include the Czech Republic, Denmark and Japan.
The top 10 regions by coal-fired electricity generation combined produce 27.7% of coal-fired electricity generation across the 37 OECD and partner countries. In these regions, owners of related capital and workers may jointly oppose a rapid coal exit and convince the regional government to do the same. Such resistance is likely to be stronger where coal use is based on local mining. Electricity generation is capital-intensive and not job-intensive. Many more jobs are at stake when electricity generation is based on local coal mines (see employment section below). Since these regions are large, they may also risk holding back zero-emission transition at higher government levels (Figure 3.21).
Figure 3.19. Most OECD countries still have at least one region with over 50% coal-fired electricity

Source: OECD calculations based on WRI (n.d.[91]), Global Power Plant Database, https://datasets.wri.org/dataset/globalpowerplantdatabase.
Figure 3.20. Coal usage for electricity generation tends to be regionally concentrated

Source: OECD calculations based on WRI (n.d.[91]), Global Power Plant Database, https://datasets.wri.org/dataset/globalpowerplantdatabase.
Figure 3.21. The 10 largest regional users of coal for electricity generation, generate over a fourth of coal-fired electricity in OECD countries

Source: OECD calculations based on WRI (n.d.[91]), Global Power Plant Database, https://datasets.wri.org/dataset/globalpowerplantdatabase.
Continued coal use can have negative impacts on coal-using and other regions. For example, air and land pollution from coal-fired power plants can be substantial and can travel far. Thermal power plants in regions subject to drought risk, which will rise with climate change in many regions, pose risks for reliable operation and aggravate risks for biodiversity and competing water use. Further risks of holding on to coal include forgoing renewable electricity production, which is sometimes already cheaper to coal, even in power plants and in the absence of adequate carbon pricing. A successful example of a coal exit in all its regions is the UK, which still produced 40% of electricity from coal in 2012. The coal exit did not generate any significant impact on the economy or electricity supply after 2012. The UK abandoned coal mining much earlier. Impacts on coal mining employment is investigated below.
Within each country, regions with more coal-fired electricity do not generally differ from regions with less coal-fired electricity in terms of GDP per capita, life satisfaction or poverty risk. Poverty risk is assessed from individuals’ survey respondents indicating there have been times in the past 12 months when they did not have enough money to buy food that they or their family needed (data on not having enough money to provide adequate shelter or housing provides similar results). Still, some regions with intensive coal use do much more poorly than their national averages, especially with respect to GDP per capita, sharply so in Colombia and Mexico (Figure 3.22). The biggest coal-using regions, particularly in Japan and the US, and regions producing all electricity with coal have mostly lower GDP per capita than their country averages.
Most regions are no longer adding or planning to add new capacity (Figures 3.23 and 3.24). Indeed, adding such capacity would expose regions to stranded asset risks, resulting in financial market risks and economic costs. Seeing that OECD regions should be phasing out coal by 2030 and the average lifespan of a coal power plant is 40 years, these planned additional generating capacities are unlikely to be aligned with the Paris Agreement. However, Australia, Colombia, Czech Republic, Greece, Japan, Korea, Mexico, Poland and Turkey still have regions where additional capacity is planned (Global Energy Monitor, 2020[92]). Carbon capture use and storage (CCUS) could mitigate related emissions, but is not being deployed at scale and would raise generation costs substantially.
Figure 3.22. GDP per capita is much lower than the national average in some regions with intensive coal use

Note: Data for 2018 or most recent year available, GDP per capita is USD per capita, PPP, prices from 2015.
Source. OECD Statistics.
Figure 3.23. Fewer OECD regions are adding new coal-fired electricity capacity

Note: This map is for illustrative purposes and is without prejudice to the status of or sovereignty over any territory covered by this map.
* New planned capacity is defined as new capacity announced, pre-permit, permit or in construction.
Source: OECD calculations based on WRI (n.d.[91]), Global Power Plant Database, https://datasets.wri.org/dataset/globalpowerplantdatabase; Global Energy Monitor (2020[92]), Global Coal Plant Tracker, https://endcoal.org/tracker/ (accessed on 16 April 2021); Source of administrative boundaries: National statistical offices, Eurostat (European Commission) © EuroGeographics and FAO Global Administrative.
Figure 3.24. Most OECD regions, especially in the Americas, are no longer adding new coal-fired electricity capacity

Note: This map is for illustrative purposes and is without prejudice to the status of or sovereignty over any territory covered by this map.
* New planned capacity is defined as new capacity announced, pre-permit, permit or in construction.
Source: OECD calculations based on WRI (n.d.[91]), Global Power Plant Database, https://datasets.wri.org/dataset/globalpowerplantdatabase; Global Energy Monitor (2020[92]), Global Coal Plant Tracker, https://endcoal.org/tracker/ (accessed on 16 April 2021); Source of administrative boundaries: National statistical offices, Eurostat (European Commission) © EuroGeographics and FAO Global Administrative.
Remote regions produce the most electricity, per capita, using renewables
As pointed out in Regions and Cities at a Glance 2020 (OECD, 2020[88]), regions located further away from metropolitan areas are, relative to their population, larger producers of electricity (Figure 3.27) and produce more from renewables. While metropolitan areas produce less than a fifth of their electricity from renewables, remote rural regions produce over half of their electricity from renewables (OECD, 2020[88]). About 80% of that comes from hydropower. However, the shares of wind and solar power in electricity production also tend to be higher in regions further away from metropolitan areas.
Regions further away from cities are generating more electricity from wind and solar, relative to their size. The generation of wind-based power is especially skewed toward most rural regions (Figure 3.28). Despite being a smaller producer of electricity overall, remote rural regions produce more wind power than large metropolitan regions and almost as much as metropolitan regions. Compared to their contribution to coal and total electricity generation, non-metropolitan regions contribute more to total wind production in the OECD. They also contribute a bigger share to solar electricity generation than to total electricity, except in remote rural regions, which also host most hydroelectric power. OECD countries still produce much more wind than solar power. With utility-scale solar photovoltaic installations continue to produce electricity at a lower cost than rooftop, the strength of solar electricity in non-metropolitan regions may continue to build up. This pattern is already marked in most of the countries with already high wind and solar shares. For example, in Spain, about three-fourths of solar is produced in non-metropolitan regions. This contrasts with the spatial distribution of nuclear or fossil-fuel-based electricity. Overall, the expansion of wind and solar electricity generation and the phase-out of coal will shift electricity generation to more rural regions.
This spatial distribution of electricity generation is likely to be reinforced as progress is made in the zero-emission transition. The IEA Sustainable Development Scenario (IEA SDS) indicates that both solar and wind electricity generation will need to increase a lot, while growth in nuclear and hydro would remain very limited. Average shares in OECD countries will need to increase to 8% and 14% by 2025 and 20% and 30% by 2040 respectively for solar and wind (Figures 3.25 and Figure 3.26). Currently, 73% of small regions (TL3) have smaller shares than the 2025 benchmark in both simultaneously. Of course, the expansion will differ across regions depending on potentials. Globally, full decarbonisation of the electricity system is required for a 1.5°C scenario (Rogejl et al., 2015[5]).
Figure 3.25. Share of solar in the energy mix, according to the Sustainable Development Scenario
Figure 3.26. Share of wind in the energy mix, according to the Sustainable Development Scenario
These trends will have regional development implications for rural regions. Wind and solar installations are land-intensive. This is one reason why curtailing energy demand growth is important. In addition, shifting electricity generation towards intermittent renewables solar and wind will require a redesign of electricity markets, making room for flexibility in demand, coupled with extensive storage and high-resolution pricing of electricity over time and space. This may reinforce the comparative advantage of high-energy-use sectors in regions with high renewables supply, including the production of hydrogen as well as the production of synthetic fuels on the basis of hydrogen, which will play a crucial role in decarbonising sectors which cannot easily be electrified, such as in industrial applications, road freight, sea and air transport. Taking advantage of intermittent renewable electricity when it is abundant and can be produced at close to zero marginal cost also requires technology adoption, such as responsive charging of electric vehicles or heat pumps. While electricity market design is a central government level task, rural and urban regions can take steps to take advantage of cheap renewable electricity when it is the most abundant, as discussed in Chapter 4.
Figure 3.27. Remote regions, relative to their population, are larger producers of electricity

Note: Data for 2017.
Source: OECD (2020[88]), OECD Regions and Cities at a Glance 2020, https://doi.org/10.1787/959d5ba0-en.
Figure 3.28. Rural regions contribute more to wind-powered electricity than large metro regions

Note: Data for 2017.
Source: OECD calculations based on WRI (n.d.[91]), Global Power Plant Database, https://datasets.wri.org/dataset/globalpowerplantdatabase.
Share of electric powered road motor vehicles
Individual passenger road transport is one of the final energy uses that will need to be decarbonised mostly through electrification, being the lowest cost option for light vehicles. According to the IEA SDS, OECD countries (European Union countries, Japan and the United States), as well as China, should fully phase out conventional car sales by 2040 (IEA, 2020[21]). The global stock of battery electric passenger vehicles should be 29 times the level it is today by 2030 (IEA, 2020[93]). To reach net-zero GHG emission targets by 2050, a phase-out date by 2035 at the latest would be needed. A more cost-effective date from the point of view of users would be 2030 (Climate Change Committee, 2019[2]), even without pencilling in the benefits of such a phase-out in terms of reduced CO2 emissions, air and noise pollution. With an average useful life of 15 years, a full phase-out would be consistent with the share of electric cars rising by 7 percentage points every year. The roll-out of charging infrastructure is key to bring this about.
Few countries currently provide data on the number of electric vehicles. Norway, which currently is the only country in the world where the majority of new vehicles sales are electric (including battery and plug-in hybrid), has the most regions with the highest share of electric passenger vehicles. Oslo and Akershus is the only large region (TL2) with over 10% (Figure 3.29). In most regions, the share is below 1%. However, Germany, the Netherlands, the UK and the US are not included in the sample. In Korea, Jeju Island has a much higher share of electric vehicles in stock than any other Korean large region (TL2). Jeju’s share grew faster because its regional government expanded its public charging infrastructure and offered incentives added to the ones provided by the national government (Kwon, Son and Jang, 2018[94]).
Electric vehicles are most common in medium-sized metropolitan areas, followed by remote rural regions. In Norway, electric cars (both full and hybrid) accounted for more than 40% of vehicle purchases in all small regions (TL3), rural and urban alike (Hall et al., 2020[95]). Electric vehicles already have lower operating costs than petrol-fired cars and purchase prices of electric vehicles are expected to fall. Reaches of new electric cars typically exceed 400 kilometres, covering almost all trips. Low operating cost makes electric cars particularly attractive for more intensive use in rural areas and shared use. As petrol tax revenues vanish, they will need to be placed with road use charging (OECD/ITF, 2019[96]). This offers the opportunity to charge higher charges in urban areas, where the external costs from vehicle use in terms of air pollution (including from tyres, also from electric vehicles), congestion and public space use are higher (OECD, 2018[97]), allowing rural regions to benefit from the low operating costs of electric vehicles.
To date, 17 countries have announced the phase-out of sales of cars with internal combustion engines (ICE) vehicles through 2050. France put this intention into law for 2040 (IEA, 2020[93]). Norway has the earliest phase-out commitment in 2025 including light vans. The UK will phase out ICE and hybrid cars by 2035. Some cities within countries with targets are setting additional targets. For example, London wants to phase out fossil fuel vehicles by 2025 (ICCT, 2020[98]).
Figure 3.29. Norway’s capital region is miles ahead of any other OECD region

Note: Selection of European countries (Austria, Hungary, Ireland, Norway, Slovak Republic and Sweden) and Korea, Mexico and Russia.
Source: OECD Statistics.
The best-documented well-being gains from the net-zero-emission transition are from lower air pollution
Policies towards net-zero GHG emissions can bring many benefits beyond halting climate change, which often accrues locally, and can therefore also serve to encourage local climate action (Box 3.8). Air pollution is among the greatest environmental health threats across the world. This is particularly true for cities, where the higher concentration of people, transport and economic activity compared to less dense areas make them more exposed to air pollution (OECD, 2020[88]; OECD, 2020[14]). The most relevant is exposure to fine particulate matter (PM2.5). Air quality is also a source of health resilience. Air pollution contributes to the airborne transmission of SARS-CoV-2 and a higher risk of mortality due to COVID-19 (Comunian et al., 2020[99]; Cole, Ozgen and Strobl, 2020[100]).
A large majority of the population in OECD countries is exposed to small particle pollution above the World Health Organization (WHO)-recommended threshold of 10 micrograms per cubic metre and virtually all populations in enhanced engagement countries (Figure 3.30). As pointed out in Regions and Cities at a Glance 2020 (OECD, 2020[88]), most cities together with their commuting zones are on average exposed to PM2.5 above the threshold. South Asian functional urban areas (FUAs) have the lowest air quality. Air pollution has been on the rise in the last 10 years in low-middle countries and has fallen little in high-income countries.
Most air pollution results from the burning of fossil fuels in transport, industry and heating. In the EU, the highest PM2.5 concentrations are measured in stations close to urban contexts and in proximity to major roads (EEA, 2020[101]). Close to half of transport emissions comes from tyre use. In some countries, agriculture and the burning of waste also contribute significantly, for example through tilling and the burning of agricultural waste. In some locations, in particular those exposed to desert winds, natural sources contribute too. Moving towards zero CO2 emissions will reduce most air pollution. Reducing the circulation of cars (especially, but not only, fuel-fired cars) can also lower energy consumption, pollution (including from vehicle tyres) and congestion, while improved agricultural practices can reduce agricultural emissions from fertilisation, both with benefits for the zero-emission-transition.
In most OECD countries, all large TL2 regions have at least 25% of the population exposed to pollution above the WHO-recommended threshold (Figure 3.31). Some more coal-dependent economies, on the other hand, have more regions exposed to air pollution. Of the 23 regions where at least some population is exposed to 3.5 times the safe level of PM2.5, 13 are Turkish. By far the worst region in terms of air quality is Chile’s most sparsely populated region Aysén. Regions with higher PM2.5 levels tend to have a lower average life satisfaction compared to their country’s average.
Box 3.8. Key local well-being benefits from a zero-emission transition
Adopting policies that are consistent with achieving the Paris Agreement objectives and prioritise health, could annually save 6.4 million lives due to healthier diets, 1.6 million lives due to cleaner air and 2.1 million lives worldwide due to increased physical activity, compared to policies that follow the NDCs, which are not yet ambitious enough to be consistent with these objectives (Hamilton et al., 2021[102]).
Reduced air pollution
Small particulate matter (PM2.5) is the biggest cause of human mortality induced by air pollution. Outdoor particulate matter causes about 422 000 premature deaths in OECD countries every year, a number which has barely fallen over the last 30 years with an equivalent estimated welfare loss of around 3% of GDP. The marginal benefit of pollution abatement on reducing mortality is high at relatively low levels around and even below the WHO-recommended threshold of 10 micrograms (Roy and Braathen, 2017[103]). For regions and cities where small particle pollution is much higher, often in middle-income countries, zero-carbon policies are therefore attractive from a local well-being-perspective, with a need to reduce pollution substantially and sustainably, with expanding economic activity.
Major disease effects include stroke, cardiovascular and respiratory disease. Air pollution amplifies respiratory infectious diseases such as COVID-19. It affects children’s health the most (WHO, 2018[104]). Education outcomes for young children exposed to higher air pollution are substantially and lastingly lower, even if exposure is temporary and even in a high-income region such as Florida in the US (Heissel, Persico and Simon, 2019[105]). Air pollution reduces worker productivity, reflecting illness and absence but perhaps also cognitive performance (Dechezleprêtre, Rivers and Stadler, 2019[106]). Worker productivity could be at least 5% higher if average exposure was below the WHO threshold: for example in Israel, productivity effects can be attributed to cognitive effects and illness. Air pollution also appears to contribute substantially to the incidence of old-age dementia (Bishop, Ketcham and Kuminoff, 2018[107]).
For instance, Schucht et al. (2015[108]) estimate at least 85% of mitigation costs are covered by co‑benefits from decreased particle and ozone levels. An empirical analysis by Chapman et al. (2018[109]) of several active travel interventions (e.g. walking and cycling) in two provincial cities in New Zealand also shows clear parallel reductions in diseases and emissions, amounting to an 11:1 benefit-cost ratio.
Reduced noise pollution
Noise pollution, especially from transport, is a growing health risk. Persistent exposure to high levels of noise can have both physical and mental health consequences. The main health threats for which causal associations have been found are cardiovascular disease, hearing and cognitive impairment, sleep disturbance, tinnitus and annoyance (WHO/JRC, 2011[110]). In addition, noise exposure has been found to affect patient outcomes and staff performance in hospitals as well as impair cognitive performance in schoolchildren (Basner et al., 2014[111]). Electric vehicles reduce noise especially at modest speeds when motor noise dominates noise from movement. Moving to active mobility and urban transport in cities can also reduce noise.
In the EU, at least 20% of the population are exposed to harmful levels of traffic noise. Chronic exposure to noise levels above the WHO standard causes 12 000 premature deaths per year worldwide and contributes to 48 000 cases of ischaemic heart disease. In addition, 6.5 million people suffer from chronic high sleep disturbance and 22 million people from “prolonged high levels of annoyance” due to noise pollution from transport or industry (EEA, 2020[112]).
Traffic congestion
The cost of traffic congestion includes time loss as well as productivity losses from higher costs in the exchange of goods and services, especially within highly productive FUAs. The productivity impact of congestion is magnified because productivity is intermediate service, affecting productivity in all sectors where transport is an input. Congestion hinders the region’s economic and social development, raises the cost of doing business and makes it harder to attain environmental goals. Costs in high-income economies are estimated: for example 1% is usually cited for the average cost of congestion in Europe and between 0.7% and 0.9% in the US. However, cities in middle-income countries appear to be substantially more congested. Congestion is increasing in most cities.
Healthier diets
The EAT-Lancet Commission on Food, Planet, Health (2018[113]) recently reported that reaching a healthy diet globally would require dividing the global consumption of red meat (beef, lamb and pork) by nearly three (more than six in North America). A change in dietary patterns would also mitigate climate change through two distinct channels: first, it would reduce direct emissions from animals; second, it would ease pressure on land use, since a large proportion of crops are grown to feed livestock. In its report on climate change and land (IPCC, 2019[8]), the IPCC estimates that changing diets have a major GHG reduction potential.
Active mobility
It is estimated that if all Londoners walked or cycled for 20 minutes a day, public health spending could be close to 0.1% of GDP lower and add 60 000 years of healthy life per year thanks to prevented illness and early death. Typically, policies that encourage active mobility also increase road safety, as it is safer roads that encourage walking and cycling.
Thermal insulation
Health benefits of building energy efficiency investment subsidies in New Zealand have been estimated to pay off the costs alone, even with high discount rates (Grimes et al., 2012[114]). In the presence of energy poverty, the health benefits of loft insulation have been estimated to exceed the costs by a multiple and be almost equal to the cost for wall insulation (Frontier Economics, 2015[115]). However, if thermal insulation employs toxic materials or leads to poor ventilation, it can also result in health costs.
Improved water, soil and biodiversity protection
Reducing fertiliser use could result in reduced nutrient runoff and water pollution, leading to healthier aquatic ecosystems. It also reduces ammonia volatilisation, which participates in the formation of particulate matters and therefore improves air quality. Low-emission farm practices which strengthen CO2 sinks also protect biodiversity.
Figure 3.30. Most population in OECD and BRIICS countries is exposed to air pollution above the WHO-recommended threshold

Note: OECD countries, Bulgaria and Romania. BRIICS: Brazil, Russia, India, Indonesia, China and South Africa.
Source: OECD Statistics.
Figure 3.31. In most OECD countries, all large regions have at least 25% of the population exposed to pollution above the WHO-recommended threshold
Employment risk from the transition appears limited
The transition to net-zero emissions will bring economic restructuring. Some sectors will shed employment. Others may be transformed substantially. This section provides an economic analysis of such risks. It uses employment data only, as regional value-added data are not available at a sufficiently detailed sectoral level. An OECD general equilibrium model, ENV-Linkages, is used to identify employment impacts across sectors of economic activity that would result from the IEA SDS, which describes an emissions pathway consistent with the Paris Agreement.
Employment data for subnational regions are only available for broad, two-digit economic sectors. The data allow identifying future regional sectoral employment in a few cases with precision but only in a few sectors, notably employment in the extraction of coal. For example, the data do not allow to distinguish employment in renewable electricity generation, which will increase, from employment in fossil fuel electricity generation, which will fall. In any case, electricity generation employs few workers. The analysis below considers employment in sectors where general equilibrium modelling suggests some employment loss will occur. These sectors may however also include activities that may not lose employment. Conversely, it may not include employment in activities that may lose employment, if these activities are in sectors in which some activities may experience employment gains (Box 3.9). Moreover, local employment risks differ depending on production costs. For example, as decarbonisation is expected to depress oil prices, production will disappear first in those regions where extraction costs are highest. In these regions, the stranded asset risk from investment in these activities is highest. Regional policymakers in member countries need more precise data to assess place-based employment at risk.
Box 3.9. The impact of the net-zero carbon transition on regional employment: Methodological approach
The selection of sectors where regions are experiencing (both direct and indirect) employment losses as economies move towards net-zero GHG emissions is based on a simulation with the OECD ENV-Linkages model. However, the sectoral employment data is only available for the large OECD TL2 regions and the sectoral data available for these regions is coarse. The selected sectors are therefore broader than those identified in ENV-Linkages.
OECD ENV-Linkages is a dynamic general equilibrium model that allows illustrating economic impacts of climate mitigation policy scenarios several decades into the future, linking activity and employment to GHG emissions (Château, Dellink and Lanzi, 2014[116]). This Regional Outlook report uses sectoral employment outcomes of a scenario that allows the goals of the Paris Agreement to be reached, following the IEA SDS. It compares employment in this scenario with employment in a baseline scenario of no further climate policies. Table 3.1 shows sectors that lose at least 3% of employment in OECD countries by 2040. This is the threshold chosen to identify sectors with regional employment risks. Employment changes in the EU (EU17) and individual economies, such as Canada, Japan and the US, are largely similar to those of the OECD as a whole. In some cases, the reduction in employment due to the zero-carbon transition is not as large for the OECD as a whole but exceeds 6% in EU17, Canada, Japan or the US. Based on this criterion, air and water transport and manufacture of other transport equipment were also included.
Matching sectoral modelling outcomes to available regional data results in a broad classification of employment risks
Regional employment data covering a selection of OECD countries is only available for broad two-digit sectors in the International Standard Industrial Classification of All Economic Activities (ISIC). These are considerably less granular than the Global Trade Analysis Project (GTAP) sectors in the ENV‑Linkages model. Unlike GTAP data, they are also not defined for the purpose of climate policy analysis.
Table 3.1. Employment changes from worldwide emission reductions consistent with the Paris Agreement, by sector
Deviation of sectoral employment from baseline in 2040, in percentages
Economic sectors |
OECD |
EU17 |
United States |
Canada |
Japan |
---|---|---|---|---|---|
Coal powered electricity |
-88 |
-89 |
-89 |
72 |
-90 |
Coal extraction |
-74 |
-80 |
-86 |
-35 |
-100 |
Gas powered electricity |
-45 |
-40 |
-41 |
-69 |
-59 |
Natural gas distribution |
-30 |
-27 |
-37 |
-49 |
-10 |
Natural gas extraction |
-30 |
-24 |
-37 |
-29 |
0 |
Oil powered electricity |
-28 |
52 |
18 |
65 |
-64 |
Petroleum and coal products |
-21 |
-32 |
-30 |
-17 |
-27 |
Electricity transmission and distribution |
-7 |
-1 |
-6 |
-17 |
-15 |
Secondary zinc, lead, gold and silver production |
-6 |
-13 |
-6 |
2 |
-9 |
Fibre crops |
-5 |
-3 |
1 |
-7 |
-4 |
Other crops (forage products, plants used in perfumery or for insecticidal purposes, etc.) |
-5 |
-7 |
1 |
-6 |
-3 |
Primary aluminium production |
-4 |
-5 |
-6 |
3 |
-7 |
Secondary aluminium production |
-4 |
-5 |
-6 |
2 |
-6 |
Primary copper production |
-4 |
-5 |
-6 |
0 |
-6 |
Primary zinc, lead, gold and silver production |
-4 |
-4 |
-6 |
1 |
-6 |
Mining of metal ores; other mining and quarrying |
-4 |
-4 |
-3 |
-7 |
-7 |
Secondary copper production |
-4 |
-4 |
-5 |
-1 |
-5 |
Textiles |
-4 |
-5 |
-3 |
-5 |
-4 |
Maize, sorghum, barley, rye, oats, millets, other cereals |
-3 |
-5 |
-3 |
-6 |
-1 |
Oil seeds and oleaginous fruit |
-3 |
-7 |
-1 |
-5 |
-1 |
Electronics |
-3 |
-1 |
-5 |
-5 |
-4 |
Chemicals, rubber, plastic products |
-3 |
-7 |
-1 |
-11 |
-1 |
Secondary iron and steel production |
3 |
8 |
2 |
4 |
3 |
Crude oil extraction |
11 |
30 |
11 |
9 |
51 |
Solar power |
74 |
69 |
142 |
61 |
70 |
Other power (biofuels, waste, geothermal, tidal technologies) |
75 |
61 |
113 |
21 |
95 |
Wind power |
78 |
45 |
141 |
39 |
323 |
Source: OECD calculations based on the ENV-Linkages model; Bibas, R., J. Chateau and E. Lanzi (2021[117]), “Policy scenarios for a transition to a more resource efficient and circular economy”, https://doi.org/10.1787/c1f3c8d0-en.
Most GTAP sectors correspond to three- or four-digit ISIC sectors, which are more granular than two‑digit sectors. The chosen two-digit ISIC sectors therefore often also include employment the modelling does not identify as being subject to employment loss. In a few cases, two-digit ISIC sectors include GTAP sectors with both gains and losses. In these cases, potential employment losses cannot be taken into account. This applies in particular to employment in fossil fuel-fired and renewable electricity generation which are part of a single two-digit ISIC sector (“electric power generation, transmission and distribution”). Moreover, sectoral employment risks can differ across regions for other reasons that cannot be taken into account. For example, climate mitigation policies should depress fossil fuel prices, resulting in the phase-out of production in those regions first where production cost is highest.
Overall, the two-digit ISIC sectors identified as being at risk of employment losses due to the net-zero carbon transition include: Mining of coal and lignite; Other mining and quarrying; Manufacture of textiles; Manufacture of coke and refined petroleum products; Manufacture of chemicals and chemical products; Manufacture of rubber and plastics products; Manufacture of other transport equipment; Water transport; Air transport. The petrochemical sectors contain most of the employment in sectors likely at risk of employment losses due to the net-zero carbon transition in OECD and partner countries: 32% of employment in sectors at risk is employed in the manufacture of rubber and plastics products and 20% is employed in the manufacture of chemicals and chemical products.
Data are available for the large regions (TL2) in 30 OECD member countries and 3 partner countries (Bulgaria, Malta and Romania). The OECD countries that are not currently included are Chile, Colombia, Iceland, Israel, Mexico, New Zealand and Turkey. For all countries except Australia and Japan, the data is for 2017. For Australia, the data is from 2019 and for Japan, the data is from 2016.
There will be both employment losses and gains due to the net-zero transition. Relative employment gains are estimated to be the largest in renewable power production and recycling of materials (Box 3.9, Table 3.1). Policies to promote renewable energy and other low-carbon activities will create demand for new service jobs (Rydge, Martin and Valero, 2018[118]). Overall, renewable energy is expected to be more employment-intensive than the fossil-fuelled energy it replaces (EC, 2018[25]). Other employment gains may come from the electrification of passenger vehicles and from early-stage innovation to diffusion for example (Unsworth et al., 2020[119]). Employment gains from the transition are difficult to project at the regional level. In any case, they may not coincide with the spatial distribution of employment losses, Employment losses are regionally concentrated and thus need place-based policies to ensure that no region is left behind. Policies to avoid regional decline and ensure a just transition for workers and local communities are discussed in Chapter 4.
Only 2.3% of employment is in sectors that are at risk of some employment loss across all countries included in the analysis. This is in line with earlier analysis, which indicates that decisive climate policy produces modest sectoral reallocation in comparison to historic sectoral job reallocation patterns (OECD, 2017[26]), although the two-digit sectoral data suggest that the Czech Republic, Germany, Korea, Poland and Switzerland might be hit slightly harder. Not a single country for which data are available has more than 4.5% of employment in sectors that on average are most at risk of employment loss. The Czech Republic, Finland, Italy, Korea, Poland and Switzerland all have at least 1 riskier region where more than 5% of employment are in sectors at risk of employment losses (Figure 3.32).
Figure 3.32. Few countries have regions with over 5% of employment in sectors at risk of employment losses due to the net-zero carbon transition
At 18%, Finland’s Åland Islands are by far the large region (TL2) with most employment in sectors at risk. This region is small in population. Italy’s Liguria and Poland’s Silesia follow with 7%. In Liguria, most are in water transport and some in the manufacture of other transport equipment. In the Polish region of Silesia, more than half of employment in sectors at risk is from employment in the mining of coal and lignite, and a quarter from employment in the manufacture of rubber and plastics products. Further, Korea’s second most populous region, Gyeongnam, and Eastern Switzerland have 6.5% of their employment in industries at risk of employment losses. For the former, about half is in the manufacture of other transport equipment, while for the latter, it is concentrated in the manufacture of chemicals, rubbers and plastics. Finally, the Czech Republic has 3 regions with over 5% of employment in industries at risk of employment losses, mostly in the manufacture of rubber and plastic products. Its Northwest region also has substantial employment in the mining of coal and lignite. Some major regions stand out compared to their national average. For example, Île-de-France has the highest employment in sectors at risk in France. This includes mostly employment in the manufacture of other transport equipment, chemicals and chemical products. The estimations consider the sectors of employment only and not the occupations of workers. Headquarters of, for example, large petrochemical companies are often in capital regions. This explains the larger measured share of employment at risk in Île-de-France. In Germany’s Rhineland-Palatinate, about 90% of employment at risk is in the manufacture of chemicals, chemical products, rubber and plastic products.
The regions with the highest shares of employment (over 4.5%) in industries at risk of employment loss are not generally regions with lower life satisfaction (Figure 3.33) or income (Annex Figure 3.A.4), nor do they significantly differ in terms of long-term unemployment rates or poverty risk, as the country notes will show. Thus the regions are not generally in a position which would make them particularly vulnerable to adverse regional developments that could result from structural change. However, some of these regions do have higher poverty risks and higher long-term unemployment and are well worth identifying. For example, for the three coal-mining regions in the Czech Republic, all are regions with a lower GDP per capita. By contrast, the Polish region has a GDP per capita higher than the national average.
Figure 3.33. Life satisfaction of regions with the highest share of employment in sectors at risk is not necessarily lower than the national average
Employment in coal mining and agriculture is often in regions with lower GDP per capita
According to the IEA Sustainable Development Scenario (SDS), coal in OECD countries will contribute less than 3% of the primary energy supply by 2040. The shares of oil and gas will also decline, though more gradually (IEA, 2020[90]). Employment in these sectors is at most about 1% of national employment. Employment in coal, oil and gas extraction and refining is above 4% in Alberta in Canada as well as in Agder and Rogaland in Norway and Wyoming in the US (Figure 3.34).
Countries analysed include Australia, Canada and the US, which are among the leading coal, oil and gas exporting countries in the world, as well as Poland. Regional employment in coal mining exceeds 1% in OECD large regions (TL2) only in the Northwest in the Czech Republic, Silesia in Poland, South-West Oltenia in Romania and West Virginia and Wyoming in the US (Figure 3.35). The Polish region of Silesia has 67 000 employees in coal mining, followed by Germany’s North-Rhine Westphalia with 13 000 workers. However, within large regions, employment at risk can be further concentrated in subregions and communities. For example, in Lesser Poland, coal mining employment is in the small region (TL3) of Oświęcimsk (ESPON, 2020[120]). Countries where regions employ more workers in coal mining have committed to later coal phase-out dates in electricity generation or have not yet committed to a phase-out (Figure 3.36).
Regions with a relatively large share of the population employed in coal mining tend to have a lower per capita GDP compared to the national average (Figure 3.37). However their position is less unfavourable when looking at household income. Indeed, the negative relation is less clear with respect to equalised disposable household income. Regions with employment in coal do not systematically differ from other regions in their respective countries in terms of average life satisfaction or long-term unemployment, though in a few countries, some regions with coal employment have more poverty, as the country notes show. Regions with oil and gas employment are not statistically different in terms of GDP per capita, relative poverty, long-term unemployment and life satisfaction.
While agriculture is not a sector that can be broadly identified as being subject to employment risks, it will be subject to important transformations, for example with respect to agricultural practices to reduce fertiliser use and carbon sequestration, including through afforestation. An avenue for climate change mitigation is from consumption habits with lower emission footprints, shifting diets away from meat and milk products of ruminant animals in high-income countries and reducing food waste (OECD, 2019[121]). These would result in health benefits but would impact related food production, especially likely so in high-cost regions. Employment in agricultural activities is very limited in OECD countries. Regions in Greece, and to a lesser extent in Australia and Finland, have higher employment shares. Ten Greek regions have over 10% of their employment in agricultural activities.1 Moreover, these regions represent 60% of its population. However, these regions also have the lowest per capita emissions related to agriculture among OECD countries with high employment in this sector. Hence, their transition risk is likely limited.
For regions where more than 2% of employment is in crop and animal production, hunting and related service activities, GDP and household income per capita tend to be lower than the national average (Figure 3.38) although the differences are not always statistically significant. Relative poverty3 is often higher in regions of Australia and Finland but not in Greece (Figure 3.39). There is no systematic difference in life satisfaction and unemployment. In any case, GHG emissions appear to be low in Greek regions but are substantial in Australian regions, suggesting that employment risks in Australia are higher.
Colombia, Ireland and New Zealand have a relatively large proportion of national employment in agricultural activities but regional employment data is not currently available. They also tend to have higher per capita emissions related to these activities, especially New Zealand – indicating a higher transition risk. Within these three countries, regions with higher agricultural emissions per capita are not poorer in terms of GDP per capita, disposable household income and relative poverty.2 However, in New Zealand in particular, regions with higher agricultural emissions per capita do tend to be poorer regions (Figure 3.40). These regions tend to have lower disposable income and higher relative poverty. In Ireland, we find that the regions with the highest emissions per capita also have a lower disposable income.
Figure 3.34. Employment in coal, oil and gas extraction and refining sectors is at most about 1% of national employment
Figure 3.35. Regional coal mining employment exceeds 1% only in Northwest Czech Republic, Silesia, South-West Oltenia, West Virginia and Wyoming
Figure 3.36. Countries with more coal mining employment have no or later coal phase-out dates in electricity generation

Source: OECD Statistics and Powering Past Coal Alliance.
Figure 3.37. Regions with the highest shares of the population employed in coal mining tend to have a lower per capita GDP compared to the national average

Note: Data for 2018 or most recent year available, no data for Bulgaria and Romania, GDP per capita is USD per capita, PPP, prices from 2015.
Source: OECD Statistics.
Figure 3.38. Some regions with over 2% employment in agriculture have lower GDP per capita than the national average

Note: Data for 2018 or most recent year available, GDP per capita is USD per capita, PPP, prices from 2015. Agriculture is defined as crop and animal production, hunting and related service activities.
Source: OECD Statistics.
Figure 3.39. Poverty is often higher in regions with over 2% of employment in agriculture in Australia and Finland but not in Greece

Note: Data for 2018. Agriculture is defined as crop and animal production, hunting and related service activities.
Source: Gallup World Poll, 2014-18.
Figure 3.40. In New Zealand in particular, regions with higher agricultural emissions per capita do tend to be poorer regions

Source: OECD calculations based on EC (2020[84]), EDGAR - Emissions Database for Global Atmospheric Research, Joint Research Centre, European Commission; Gallup World Poll, 2014-18; OECD Statistics.
Rural regions and poorer metropolitan areas are more car-dependent
Transitioning to a net-zero GHG emission economy requires a shift away from fossil-fuel-powered cars. Governments may decide to implement policies to move to zero-carbon cars and reduce car use. The latter can offer additional benefits, notably lower energy consumption, a bigger reduction in air pollution, lower traffic congestion, more room for active mobility and other use of urban space taken up by cars. Regions with high use of fuel-powered cars are more sensitive to potential costs and perhaps also to the benefits. Traffic congestion, air pollution from traffic and space constraints are less important in remote rural regions, however. More rural regions tend to be more car-dependent (Figure 3.41). Policies to price fossil-fuel-fired cars out of use would have strong welfare consequences among car-dependent communities, where the elasticity of car use with respect to the price is low. Compensation would have to be precisely targeted but the low operating cost of electric cars can make their phase-in attractive.
Figure 3.41. Rural regions are more car-dependent

Note: Latest available data year from 2010 onwards, for 24 OECD countries. Definitions of private vehicles differ across countries. For example, the EU defines passenger vehicles as vehicles “designed...for the carriage of passengers and not exceeding eight seats”. The US, on the other hand, defines passenger vehicles primarily based on weight. Consequently, sport utility vehicles (SUVs) are not classified as passenger vehicles, although they are often used this way in the US. Hence, if it were included, US rates would be higher.
Source: OECD Statistics.
Public transport performance needs large improvements to encourage a modal shift
A shift to public transport and active mobility needs to be part of the net-zero GHG emission transition. Additional to electrifying light transport in general, a shift away from cars towards public transport and active mobility can ease the transition by reducing energy demand and the infrastructure needs related to electrification. It can also provide substantial well-being benefits from reduced congestion, air and noise pollution, increased safety and public space, as well as health benefits from active mobility. It can even reduce pollution when road transport is entirely decarbonised, as particles of rubber from tyres are currently responsible for nearly half of road transport particulate emissions.
Across countries, public transport performance is typically higher in European and South American FUAs, which include cities and their surrounding commuting areas (OECD/EC, 2020[40]). Public transport performance measures how well it gets people to destinations (Box 3.10). Within-country comparison of cities is currently only possible for Europe and to some extent North America (Figure 3.42). In Europe, variances in performance within-country are large, especially in the UK. This may allow setting benchmarks and improve performance in cities with poor public transport performance.
Figure 3.42. Where within-country comparison is possible, regional differences in public transport performance are clearly large

Sources: OECD Statistics and International Transport Forum.
Box 3.10. Defining public transport performance
Public transport performance measures the ratio between accessible destinations within a certain distance and nearby destinations for different transport modes within a set period of time. The destinations can be facilities, schools, hospitals or other people. In this report, the chosen destination is other people. The indicator captures many aspects of the effectiveness of a transport mode in providing access to destinations. For example, public transport performance depends among other things on how many people live close to a stop, the frequency and the speed of public transport vehicles and the design of the network (ITF, 2019[122]). Analysis in this section is based on 30 min/8 km. The index reaches one when all destination within 8 km can be reached within 30 minutes. When more people can be reached than are proximate, the ratio will be larger than one. If fewer people can be reached in 30 minutes than are proximate in an 8 km radius, the ratio will be between 0 and 1.
Smaller cities are less prepared to follow a decarbonisation pathway via modal shift. Public transport performance tends to be higher for larger cities, in terms of population, in each country. This is especially true for most of the large capital cities. While mass transit can be deployed with more frequent service and lower cost in denser cities (ITF, 2019[122]), currently, denser cities are not more likely to have a higher public transport performance in all countries. Cities with a lower GDP per capita tend to have a worse public transport performance score in some countries, such as in France and the UK, and are thus more car-dependent. This suggests national policies are needed to improve public transport in the poorest cities. It may also reflect higher productivity performance in cities as a result of better public transport performance (Figure 3.43).
FUAs with better accessibility by car than by public transport are likely to need more investment to achieve modal shift. For all FUAs except London, accessibility is better by car than by public transport (Figure 3.44). For the vast majority, taking the car even offers twice the level of accessibility. London has both the best public transport and worst car performance accessibility in Europe. This is a consequence of legacy decisions against building express roads through central London and more recently deliberate policies to reallocate road space to public transport and cycling (ITF, 2019[122]). London also has developed a system to measure the accessibility of residents to jobs and key facilities, so transport infrastructure and urban planning can serve to improve accessibility, as well as a congestion charge. London’s performance notwithstanding, generally public transport performs particularly poorly compared to cars (Figure 3.44).
Figure 3.43. Cities with a lower GDP per capita tend to have worse public transport performance
Road freight hubs need to engage with the zero-carbon transition
Heavy-duty road freight accounts for 22% of transport-related CO2 emissions and over 5% of total energy-related CO2 emissions worldwide. Decarbonisation in road freight is less advanced than passenger transport, as zero-carbon technologies to be deployed at scale have not yet been chosen. However, deployment of such technologies is a near-term priority to move road freight towards net-zero emissions (IEA, 2017[123]).
Railways and inland waterways could take a larger share but the infrastructure is not always in place and it is costly and not always feasible to build (IEA, 2020[21]). For example, the EU aims to shift 50% of medium-distance freight journeys to rail by 2050. Better multimodal solutions for long-distance transport of goods are needed (EC, 2018[124]). In any case, a segment of the deliveries (e.g. port to road, road to rail) almost always requires road haulage. To decarbonise these, zero-emission technologies need to be deployed, going beyond battery electric vehicles, which may not be suitable for heavy loads. These could be hydrogen and synthetic fuels. Infrastructure investments by governments and industry are needed with synergies for battery electric and hydrogen fuel cell buses (ICCT, 2017[125]). The majority of alternative fuels require new distribution networks and refuelling or recharging stations. All of these bring challenges for infrastructure supply and management (ITF, 2018[126]).
Figure 3.44. Public transport performs poorly compared to cars

Note: The difference in transport performance is calculated as the car transport performance score minus the public transport performance score.
Sources: OECD Statistics and ITF.
Regional challenges from freight transport decarbonisation include these large infrastructure investments. Some standardisation of truck technology and infrastructure systems (for electric vehicles that are dynamically charged) across regions might be necessary for long international routes (ICCT, 2017[125]). In the near term, reducing emissions from trucking will require systemic improvements in supply chains, logistics and routing, supported by new technologies, improved vehicle utilisation, backhauling, last-mile efficiency measures and re-timing urban deliveries. Regulation will need to enable many of these systemic changes with substantial efficiency gains (IEA, 2017[123]). Finally, there will likely be net employment and gross value-added gains as well as changes in qualification requirements and wages when shifting from road to rail and possibly waterborne transport, as well as towards zero-carbon technologies. But overall, the economic and labour market impacts are small (Doll et al., 2019[127]).
The introduction of new logistics, technologies as well as the shift to multimodal transport may particularly affect regions with large volumes of transport loading and unloading. The three regions with the highest tonnage of freight loading are Spanish – Barcelona, Madrid and Valencia – followed by Sweden’s second-largest county Västra Götalands län, the major port city in northern Germany Hamburg and regions in France (Figure 3.45). Most goods are loaded in intermediate to urban areas (Figure 3.46).
Climate models provide insights into future regional hazards
High-resolution climate models can provide indicators of climate hazard at regional and local scales that can contribute to preventive adaptation and build a more resilient society, for different global warming or emission scenarios. The following section illustrates place-based modelling results and their use to integrate adaptation in regional development policy. Spatial variations in climate hazards are substantial even within subnational regions. But there are also substantial variations across models and scenarios especially for projected climate hazards (Schoof and Robeson, 2016[128]). Ensemble of models can improve the robustness of the results but may also risk averaging out extreme outcomes.
Figure 3.45. Spanish regions have the highest tonnage of road freight loading
Figure 3.46. Most road freight goods are loaded in intermediate to urban areas
Climate hazards in European regions
Climate-induced hazards including heatwaves, cold waves, river and coastal floods, wildfires, droughts and windstorms. Windstorms and flood are dominant hazards today but, in the future, drought and heatwaves will dominate. Under a “business as usual” scenario (no further climate change mitigation), they are expected to rise tenfold with significant spatial variations across Europe (Figure 3.47).
Figure 3.47. Cost of multi-hazard damages across Europe to 2080 assuming no further climate change mitigation action

Note: EAD denotes expected annual damage over the period under consideration. Multi-hazard includes heatwaves, cold waves, floods, wildfires, droughts and windstorms. Hazard and associated losses were obtained from the Emergency Events Database. Climate scenario SRES–A1B consistent with “business as usual”.
Source: Forzieri, G. et al. (2018[38]), “Escalating impacts of climate extremes on critical infrastructures in Europe”, https://doi.org/10.1016/j.gloenvcha.2017.11.007.
Flood hazard in Japan
Japan has a long history of typhoons, flooding and heavy rainfall and has a well-formulated flood risk reduction strategy (Fan and Huang, 2020[129]). Flood fatalities trend downward in Japan. Nonetheless, 50% of the population and 75% of national assets are concentrated in flood-prone areas (Fan and Huang, 2020[129]). Flooding from extreme rainfall is expected to increase and inflict economic losses. Precipitation is expected to increase significantly with large regional differences (Tezuka et al., 2014[130]). One finding of this modelling is that the flood protection suitable for floods of a strength that may only occur every 50 years will only protect against floods that are likely to return every 30 years by 2050.
Climate hazard across Australia
Global warming has inflicted longer periods of drought, heatwaves, wildfires or bushfires. The likelihood of fire risk is increasing with rising temperature and heatwaves. Heatwaves are the deadliest natural hazard with detrimental impacts on coral reefs, native fauna, human health, infrastructure and agriculture (Alexandra, 2020[131]). They are projected to triple in the future with spatial variation between regions and cities measured in terms of different heatwave indices (Herold et al., 2018[132]) (Figure 3.48).
Figure 3.48. Heatwaves across Australia relative to recent past

Note: HWD in days, HWM expressed as excess heat in ℃. SRES A2 scenario consistent with about 3.5-degree global warming by 2100.
Source: Herold, N. et al. (2018[132]), “Australian climate extremes in the 21st century according to a regional climate model ensemble: Implications for health and agriculture”, https://doi.org/10.1016/j.wace.2018.01.001.
Climate hazard in the US
In the US, hurricanes are the most destructive climate hazard. Hurricanes have increased in intensity as well as in the frequency of occurrence between 1900 and 2018, which is attributed to global warming (Grinsted, Ditlevsen and Christensen, 2019[133]). Global warming will increase storm tide heights. Coastal floods are hence expected to increase, which will require a combination of several adaptation measures such as armouring floodwalls, elevating structures, shoreline nourishment (increasing amount of sand to replenish beach profile) and abandoning property (Lorie et al., 2020[134]). Scenarios of sea level rise at 0.5 m, 1 m, 1.5 m and 2 m by 2100 were assessed for the coastal regions of Tampa, Florida, for example. For the case of Pinellas County in Tampa, a combination of the four adaptation approaches is expected to minimise economic damage and loss of human lives (Figure 3.49). This exercise can illustrate how regional policymakers can work with modellers. They can provide policy questions as inputs to deploy modelling work, which can protect valuable local physical and social infrastructure, drawing on local knowledge.
Figure 3.49. Projected mix of adaptation approaches under sea level rise in Pinellas County, Florida, US

Source: Lorie, M. et al. (2020[134]), “Modeling coastal flood risk and adaptation response under future climate conditions”, https://doi.org/10.1016/j.crm.2020.100233.
Climate impacts include the number of days with minimum temperature above 20°C (Schoof and Robeson, 2016[128]) indicating more chances of heatwaves. Also, there will be a net reduction in agricultural yields by 9% per 1°C rise in warming after accounting for yield increase from CO2 concentration in cold places (Hsiang et al., 2017[47]).
Road infrastructure damage in Mexico
Mexico is vulnerable to drought as most of its territory is arid or semi-arid (Soto-Montes-de-Oca and Alfie-Cohen, 2019[135]). The drought risk is expected to increase. For example, by 2080 (in a scenario consistent with global warming of 2°C), dry events will increase by 175%, whereas wet periods will decrease by 86%, aggravating scarcity of water (Herrera-Pantoja and Hiscock, 2015[136]). Some local communities will need to anticipate human and livestock morbidity, discomfort in working outside and the likelihood of abandoning agriculture (Soto-Montes-de-Oca and Alfie-Cohen, 2019[135]).
Changing temperature and precipitation are also projected to inflict damage to the road infrastructure. The cost of repairing and maintaining roads’ functionality may range between USD 1.3 billion to 4.8 billion at the national level during the period 2015-50 (Figure 3.50). These costs vary from 1% of road inventory for the least vulnerable state to 100% for the most vulnerable state under severe climatic change. The modelling work provided vital information such as in terms of the future cost of damage on road networks, which can inform science-based decision-making to integrate adaptation actions in road infrastructure planning.
Figure 3.50. Climate-change-induced road maintenance costs vary strongly across Mexican regions

Note: Scenario corresponds to 2℃ warming by the end of the 21st century.
Source: Espinet, X. et al. (2016[137]), “Planning resilient roads for the future environment and climate change: Quantifying the vulnerability of the primary transport infrastructure system”, https://doi.org/10.1016/j.tranpol.2016.06.003.
Summing up: Policy conclusions from Chapter 3
Climate change is a global challenge requiring local action. Reaching the objectives of the Paris Agreement will prevent major threats to the foundations of human well-being. These are substantially worse under 2°C of global warming than under 1.5°C. Most OECD countries, therefore, aim at reaching net-zero domestic GHG emissions in 2050. Deep transformations of unprecedented breadth over a short period of time are needed to reach this target. There is a need for place-based policies that align with national and global objectives to address climate change mitigation and adaptation.
Well-being benefits beyond the protection of the climate can more than offset the cost of climate action in many regions. A large majority of the population in OECD regions and cities is exposed to unhealthy levels of small particulate pollution, with negative effects on mortality, disease, vulnerability to COVID-19, child health, education and worker productivity. Other well-being gains include reduced noise pollution and traffic congestion, healthier diets, enhanced health due to increased active mobility, health benefits through thermal insulation and improved water, soil and biodiversity protection. Negative GDP effects of the transition are more marked in fossil-fuel exporting regions. Some regions with intensive coal use do much more poorly than their national averages, especially with respect to GDP per capita, and need support not to be left behind.
Delayed action raises costs substantially, including in the cities and regions where they occur. Anticipation and support for vulnerable local populations are critical. Anticipation prevents potentially disastrous climate impacts which hit the vulnerable the most in their livelihoods. Adequate income and access to key services, such as healthcare and water, are key for the vulnerable to be resilient to climate-change-related impacts.
Subnational governments need to play a key role in the net-zero transition:
Local conditions are critical for defining net-zero-emission strategies, for example for connecting people to jobs, for the specific industrial mix and enterprise fabric of cities and regions.
Subnational governments have key relevant competencies for climate policy. The three pillars of climate mitigation action – energy, land use, urban policy – are at the heart of regional development. Local and regional governments play an essential role in supporting the most vulnerable as they understand the local issues.
Governments at all levels should assess all their investment decisions against the net-zero-emission transition. Some regions heavily invested in fossil fuel extraction and transformation are particularly at risk from losses if investment in these activities continues.
Well-being benefits beyond climate often arise regionally in the near term and require local and regional action to harness them.
The impacts, exposures and vulnerabilities to climate change differ across locations and need to be identified and addressed locally and regionally.
Indicators to measure regional progress on the net-zero-emission transition have shown that:
GHG emissions vary hugely across territories and degree of rurality:
Regions with different production-based emissions will have different transition pathways.
While metropolitan regions contribute most to total GHG emissions (about 60%), rural regions’ emissions per capita are higher.
Within-country variation in emissions is higher than between countries.
Regions with higher production-based emissions per capita tend to have higher GDP per capita.
Most OECD countries still have regions that rely on coal-fired electricity generation:
Coal in electricity generation should be largely phased out by 2030 but the transition to zero-carbon electricity remains unequal across regions.
Some regions in Australia, Colombia, Greece, Japan, Korea, Poland and Turkey are still planning or adding new coal-fired electricity generation capacity, which exposes them to losses on the investment.
Regions need to move more decisively to renewables:
Remote regions produce the most electricity, per capita, using renewables. The generation of wind-based power is especially skewed toward most rural regions.
Overall, the expansion of wind and solar electricity generation and the phase-out of coal will shift electricity generation to more rural regions as progress is made in the zero-emission transition. These trends will have regional development implications for rural regions.
While electricity market design is a central government level task, rural and urban regions can take steps to take advantage of cheap renewable electricity when it is the most abundant, as discussed in Chapter 4.
Electric cars, public transport and active mobility need to grow more rapidly:
A cost-effective date for phasing out the sale of new fossil-powered cars is 2030.
Regional governments can encourage electric vehicle uptake by expanding public charging infrastructure and offering additional incentives. Shifting mobility towards public transport and active mobility can ease the transition by reducing transport energy demand as well as infrastructure and material needs. Policies to encourage such a shift are described in Chapter 4.
These indicators are also examined for each country in the online country notes. However, across the OECD, regional data to measure transition progress is lacking. For example, regional progress in making energy use in all buildings consistent with net-zero emissions and required renovations cannot be tracked.
Employment losses from the transition appear limited:
The employment risks are modest compared to historical job allocation and anticipated restructuring due to digitalisation. On average, 2.3% of employment is at risk in OECD regions.
Job losses are regionally concentrated. Some of the regions with over 5% of employment at risk have higher poverty risks and higher long-term unemployment. Polices to ensure that no region is left behind are further discussed in Chapter 4.
There will also be employment gains from the transition.
Place-based adaptation needs to complement decisive mitigation:
Regional policymakers can work with modellers to identify exposures and vulnerabilities to protect valuable local physical and social infrastructure, drawing on local knowledge.
Annex 3.A. Annex charts
Annex Figure 3.A.1. Regional emissions per capita and GDP per capita are positively correlated

Source: OECD calculations based on EC (2020[84]), EDGAR - Emissions Database for Global Atmospheric Research, Joint Research Centre, European Commission; OECD Statistics.
Annex Figure 3.A.2. In some top-emitting regions, GDP per capita is very high with little difference in life satisfaction

Note: Panel B: No data for Canada and Spain.
Source: Panel A: OECD Statistics; Panel B: Gallup World Poll, 2014-18.
Annex Figure 3.A.3. Difference between regional life satisfaction and national average for regions with largest coal-fired electricity production

Source: Gallup World Poll, 2014-18.
Annex Figure 3.A.4. Difference between regional GDP per capita and the national average for TL2 regions with highest shares of employment in sectors with employment at risks

Source: OECD Statistics.
References
[69] Ainsworth, G. et al. (2020), “Integrating scientific and local knowledge to address conservation conflicts: Towards a practical framework based on lessons learned from a Scottish case study”, Environmental Science & Policy, Vol. 107, pp. 46-55, https://doi.org/10.1016/j.envsci.2020.02.017.
[131] Alexandra, J. (2020), “Burning bush and disaster justice in Victoria, Australia: Can regional planning prevent bushfires becoming disasters?”, in Lukasiewicz, A. and C. Baldwin (eds.), Natural Hazards and Disaster Justice: Challenges for Australia and Its Neighbours, Springer Singapore Pte. Limited, https://doi.org/10.1007/978-981-15-0466-2_4.
[53] Alves, A. et al. (2019), “Assessing the co-benefits of green-blue-grey infrastructure for sustainable urban flood risk management”, Journal of Environmental Management, Vol. 239, pp. 244-254, https://doi.org/10.1016/j.jenvman.2019.03.036.
[111] Basner, M. et al. (2014), “Auditory and non-auditory effects of noise on health”, The Lancet, Vol. 383/9925, pp. 1325-1332, http://dx.doi.org/10.1016/s0140-6736(13)61613-x.
[117] Bibas, R., J. Chateau and E. Lanzi (2021), “Policy scenarios for a transition to a more resource efficient and circular economy”, OECD Environment Working Papers, No. 169, OECD Publishing, Paris, https://doi.org/10.1787/c1f3c8d0-en.
[107] Bishop, K., J. Ketcham and N. Kuminoff (2018), Hazed and Confused: The Effect of Air Pollution on Dementia, National Bureau of Economic Research, Cambridge, MA, http://dx.doi.org/10.3386/w24970.
[13] Chapman, R. (2019), “Managing the transition to a climate-neutral economy in cities and regions”, Background paper for an OECD/EC workshop on 17 May 2019 within the workshop series “Managing environmental and energy transitions for regions and cities”, Paris.
[109] Chapman, R. et al. (2018), “A cost benefit analysis of an active travel intervention with health and carbon emission reduction benefits”, International Journal of Environmental Research and Public Health, Vol. 15/5, p. 962.
[116] Château, J., R. Dellink and E. Lanzi (2014), “An Overview of the OECD ENV-Linkages Model: Version 3”, OECD Environment Working Papers, No. 65, OECD Publishing, Paris, https://dx.doi.org/10.1787/5jz2qck2b2vd-en.
[89] Climate Analytics (2019), Global and Regional Coal Phase-out Requirements of the Paris Agreement: Insights from the IPCC Special Report on 1.5°C.
[3] Climate Analytics/New Climate Institute (n.d.), Climate Action Tracker, https://climateactiontracker.org/ (accessed on September 2020).
[2] Climate Change Committee (2019), Net Zero - The UK’s Contribution to Stopping Global Warming, Climate Change Committee, https://www.theccc.org.uk/publication/net-zero-the-uks-contribution-to-stopping-global-warming/ (accessed on 23 October 2019).
[100] Cole, M., C. Ozgen and E. Strobl (2020), “Air pollution exposure and Covid-19 in Dutch municipalities”, Environmental and Resource Economics, Vol. 76/4, pp. 581-610, https://doi.org/10.1007/s10640-020-00491-4.
[76] Colvin, R. et al. (2020), “Implications of climate change for future disasters”, in Lukasiewicz, A. and C. Baldwin (eds.), Natural Hazards and Disaster Justice: Challenges for Australia and Its Neighbours, Springer Singapore Pte. Limited, https://doi.org/10.1007/978-981-15-0466-2_2.
[99] Comunian, S. et al. (2020), “Air pollution and Covid-19: The role of particulate matter in the spread and increase of Covid-19’s morbidity and mortality”, International Journal of Environmental Research and Public Health, Vol. 17/12, p. 4487.
[86] Crippa, M. et al. (2020), Fossil CO2 Emissions of All World Countries - 2020 Report, EUR 30358 EN, Publications Office of the European Union, Luxembourg, http://dx.doi.org/10.2760/143674.
[79] Cutter, S. (2018), “Compound, cascading, or complex disasters: What’s in a name?”, Environment: Science and Policy for Sustainable Development, Vol. 60/6, pp. 16-25, http://dx.doi.org/10.1080/00139157.2018.1517518.
[27] Dechezleprêtre, A. (2019), “The economic cost of air pollution: Evidence from Europe”.
[106] Dechezleprêtre, A., N. Rivers and B. Stadler (2019), “The economic cost of air pollution: Evidence from Europe”, OECD Economics Department Working Papers, No. 1584, OECD Publishing, Paris, https://dx.doi.org/10.1787/56119490-en.
[82] Dodman, D. et al. (2019), “A spectrum of methods for a spectrum of risk: Generating evidence to understand and reduce urban risk in sub-Saharan Africa”, Area, Vol. 51/3, pp. 586-594, http://dx.doi.org/10.1111/area.12510.
[127] Doll, K. et al. (2019), From Local to European Low Emission Freight Concepts, Summary Report 3: European Rail Freight Corridors for Europe, Fraunhofer Institute for Systems and Innovation Research ISI.
[62] Dolšak, N. and A. Prakash (2018), “The politics of climate change adaptation”, Annual Review of Environment and Resources, Vol. 43/1, pp. 317-341, http://dx.doi.org/10.1146/annurev-environ-102017-025739.
[113] EAT-Lancet Commission (2018), Food Planet Health: Healthy Diets From Sustainable Food Systems, Summary Report of the EAT-Lancet Commission, https://eatforum.org/content/uploads/2019/01/EAT-Lancet_Commission_Summary_Report.pdf.
[84] EC (2020), EDGAR - Emissions Database for Global Atmospheric Research, Joint Research Centre, European Commission.
[58] EC (2019), Review of Progress on Implementation of the EU Green Infrastructure Strategy: Report from the Commission to the European Parliament, the Council, the European Economic and Social Committee and the Committee of the Regions, European Commission.
[25] EC (2018), “A clean planet for all: A European long-term strategic vision for a prosperous, modern, competitive and climate neutral economy”, No. COM(2018) 773, In-depth Analysis in Support of the Commission Communication, European Commission.
[124] EC (2018), Final Report of the High-Level Panel of the European Decarbonisation Pathways Initiative, European Commission.
[83] Edwards, C. (2009), Resilient Nation, http://www.continuitycentral.com/ResilientNation.pdf.
[101] EEA (2020), Air Quality in Europe, 2020 Report, European Environment Agency.
[112] EEA (2020), Environmental Noise in Europe - 2020, European Environment Agency, http://dx.doi.org/10.2800/686249.
[54] Emmanuel, R. and A. Loconsole (2015), “Green infrastructure as an adaptation approach to tackling urban overheating in the Glasgow Clyde Valley Region, UK”, Landscape and Urban Planning, Vol. 138, pp. 71-86, https://doi.org/10.1016/j.landurbplan.2015.02.012.
[137] Espinet, X. et al. (2016), “Planning resilient roads for the future environment and climate change: Quantifying the vulnerability of the primary transport infrastructure system”, Transport Policy, Vol. 50, pp. 78-86, https://doi.org/10.1016/j.tranpol.2016.06.003.
[120] ESPON (2020), Policy Brief: Structural Change in Coal Phase-out Regions.
[129] Fan, J. and G. Huang (2020), “Evaluation of flood risk management in Japan through a recent case”, Sustainability, Vol. 12/13, p. 17, http://dx.doi.org/10.3390/su12135357.
[68] Flores, C. et al. (2019), “Governance assessment of the flood’s infrastructure policy in San Pedro Cholula, Mexico: Potential for a leapfrog to water sensitive”, Sustainability, Vol. 11/24, http://dx.doi.org/10.3390/su11247144.
[37] Formetta, G. and L. Feyen (2019), “Empirical evidence of declining global vulnerability to climate-related hazards”, Global Environmental Change, Vol. 57, https://doi.org/10.1016/j.gloenvcha.2019.05.004.
[38] Forzieri, G. et al. (2018), “Escalating impacts of climate extremes on critical infrastructures in Europe”, Global Environmental Change, Vol. 48, https://doi.org/10.1016/j.gloenvcha.2017.11.007.
[12] Fragkos, P. (2020), “Global energy system transformations to 1.5°C: The impact of revised Intergovernmental Panel on Climate Change carbon budgets”, Energy Technology, Vol. 8/9, p. 2000395, https://doi.org/10.1002/ente.202000395.
[115] Frontier Economics (2015), Energy efficiency: An infrastructure priority, https://www.frontier-economics.com/media/1009/20150923_energy-efficiency_an-infrastructure-priority_frontier.pdf (accessed on 15 October 2020).
[5] Garbe, J. et al. (2020), “The hysteresis of the Antarctic Ice Sheet”, Nature, Vol. 585/7826, pp. 538-544, http://dx.doi.org/10.1038/s41586-020-2727-5.
[44] Global Commission on Adaptation (2019), Adapt Now: A Global Call for Leadership on Climate Resilience, Global Centre on Adaptation and World Resource Institute, https://gca.org/reports/adapt-now-a-global-call-for-leadership-on-climate-resilience/.
[92] Global Energy Monitor (2020), Global Coal Plant Tracker, https://endcoal.org/tracker/ (accessed on 7 October 2020).
[63] Grecksch, K. and C. Klöck (2020), “Access and allocation in climate change adaptation”, International Environmental Agreements: Politics, Law and Economics, Vol. 20/2, pp. 271-286, http://dx.doi.org/10.1007/s10784-020-09477-5.
[59] Greenhill, B., N. Dolšak and A. Prakash (2018), “Exploring the adaptation-mitigation relationship: Does information on the costs of adapting to climate change influence support for mitigation?”, Environmental Communication, Vol. 12/7, pp. 911-927, http://dx.doi.org/10.1080/17524032.2018.1508046.
[71] GRI (2019), UK Business Adaptation Survey, Global Reporting Initiative.
[114] Grimes, A. et al. (2012), Cost Benefit Analysis of the Warm Up New Zealand: Heat Smart Programme, Report to the Ministry of Economic Development.
[133] Grinsted, A., P. Ditlevsen and J. Christensen (2019), “Normalized US hurricane damage estimates using area of total destruction, 1900-2018”, Proceedings of the National Academy of Sciences, Vol. 116/48, http://dx.doi.org/10.1073/pnas.1912277116.
[87] Gurney, K. et al. (2021), “Under-reporting of greenhouse gas emissions in U.S. cities”, Nature Communications, Vol. 12/1, pp. 1-7.
[15] Haberl, H. et al. (2020), “A systematic review of the evidence on decoupling of GDP, resource use and GHG emissions, part II: Synthesizing the insights”, Environmental Research Letters, Vol. 15/6, http://dx.doi.org/10.1088/1748-9326.
[95] Hall, D. et al. (2020), European Electric Vehicle Factbook 2019/2020, International Council on Clean Transportation, https://theicct.org/publications/european-electric-vehicle-factbook-20192020.
[41] Hallegatte, S. et al. (2013), “Future flood losses in major coastal cities”, Nature Climate Change, Vol. 3/9, pp. 802-806, http://dx.doi.org/10.1038/nclimate1979.
[102] Hamilton, I. et al. (2021), “The public health implications of the Paris Agreement: A modelling study”, The Lancet Planetary Health, Vol. 5/2, pp. e74-e83, http://dx.doi.org/10.1016/s2542-5196(20)30249-7.
[105] Heissel, J., C. Persico and D. Simon (2019), Does Pollution Drive Achievement? The Effect of Traffic Pollution on Academic Performance, National Bureau of Economic Research, Cambridge, MA, http://dx.doi.org/10.3386/w25489.
[132] Herold, N. et al. (2018), “Australian climate extremes in the 21st century according to a regional climate model ensemble: Implications for health and agriculture”, Weather and Climate Extremes, Vol. 20, pp. 54-68, https://doi.org/10.1016/j.wace.2018.01.001.
[136] Herrera-Pantoja, M. and K. Hiscock (2015), “Projected impacts of climate change on water availability indicators in a semi-arid region of central Mexico”, Environmental Science & Policy, Vol. 54, pp. 81-89, https://doi.org/10.1016/j.envsci.2015.06.020.
[55] Hiscock, R. et al. (2017), “City scale climate change policies: Do they matter for wellbeing?”, Preventive Medicine Reports, Vol. 6, pp. 265-270, http://dx.doi.org/10.1016/j.pmedr.2017.03.019.
[48] Hsiang, S. and M. Burke (2014), “Climate, conflict, and social stability: What does the evidence say?”, Climatic Change, Vol. 123/1, pp. 39-55, http://dx.doi.org/10.1007/s10584-013-0868-3.
[47] Hsiang, S. et al. (2017), “Estimating economic damage from climate change in the United States”, Science, Vol. 356/6345, http://dx.doi.org/10.1126/science.aal4369.
[98] ICCT (2020), “The end of the road? An overview of combustion-engine car phase-out announcements across Europe”, International Council on Clean Transportation.
[125] ICCT (2017), “Transitioning to zero-emission heavy-duty freight vehicles”, International Council on Clean Transportation.
[21] IEA (2020), Energy Technology Perspectives 2020, OECD Publishing, Paris, https://dx.doi.org/10.1787/d07136f0-en.
[93] IEA (2020), Global EV Outlook 2020: Entering the Decade of Electric Drive?, OECD Publishing, Paris, https://dx.doi.org/10.1787/d394399e-en.
[90] IEA (2020), World Energy Outlook 2020, OECD Publishing, Paris, https://dx.doi.org/10.1787/557a761b-en.
[19] IEA (2019), Global Energy & CO2 Status Report 2019, IEA, Paris, https://www.iea.org/reports/global-energy-co2-status-report-2019/emissions (accessed on 3 April 2020).
[33] IEA (2019), World Energy Outlook 2019, IEA, Paris, https://www.iea.org/reports/world-energy-outlook-2019 (accessed on 3 April 2020).
[123] IEA (2017), Energy Technology Perspectives 2017: Catalysing Energy Technology Transformations, International Energy Agency, Paris, https://dx.doi.org/10.1787/energy_tech-2017-en.
[17] IEA (n.d.), World Energy Balances, International Energy Agency.
[22] IPCC (2019), Climate Change and Land, Intergovernmental Panel on Climate Change.
[8] IPCC (2019), Climate Change and Land - Summary for Policymakers, IPCC Special Report on Climate Change, Desertification, Land Degradation, Sustainable Land Management, Food Security, and Greenhouse Gas Fluxes in Terrestrial Ecosystems, Intergovernmental Panel on Climate Change, https://www.ipcc.ch/site/assets/uploads/2019/08/4.-SPM_Approved_Microsite_FINAL.pdf.
[4] IPCC (2018), Global Warming of 1.5 ºC, Intergovernmental Panel on Climate Change, https://www.ipcc.ch/sr15/ (accessed on 13 May 2020).
[11] IPCC (2018), Global warming of 1.5°C - Summary for Policymakers, Intergovernmental Panel on Climate Change, http://report.ipcc.ch/sr15/pdf/sr15_spm_final.pdf.
[65] IPCC (2014), Climate Change 2014: Synthesis Report, Contribution of Working Groups I, II and III to the Fifth Assessment Report of the Intergovernmental Panel on Climate Change.
[60] IPCC (2012), Managing the Risks of Extreme Events and Disasters to Advance Climate Change Adaptation. Special Report of Working Groups I and II of the Intergovernmental Panel on Climate Change, Intergovernmental Panel on Climate Change, Cambridge University Press, https://www.ipcc.ch/report/managing-the-risks-of-extreme-events-and-disasters-to-advance-climate-change-adaptation/.
[20] IRENA (2020), Global Renewables Outlook: Energy Transformation 2050, International Renewable Energy Agency, https://www.irena.org/publications/2020/Apr/Global-Renewables-Outlook-2020 (accessed on 17 July 2020).
[122] ITF (2019), “Benchmarking Accessibility in Cities: Measuring the Impact of Proximity and Transport Performance”, International Transport Forum Policy Papers, No. 68, OECD Publishing, Paris, https://doi.org/10.1787/4b1f722b-en.
[126] ITF (2018), “Towards Road Freight Decarbonisation: Trends, Measures and Policies”, International Transport Forum Policy Papers, No. 64, OECD Publishing, Paris, https://doi.org/10.1787/3dc0b429-en.
[85] Janssens-Maenhout, G. et al. (2019), “EDGAR v4. 3.2 Global Atlas of the three major greenhouse gas emissions for the period 1970-2012”, Earth System Science Data, Vol. 11/3, pp. 959-1002.
[28] Karlsson, M., E. Alfredsson and N. Westling (2020), “Climate policy co-benefits: A review”, Climate Policy, Vol. 20/3, pp. 292-316, https://doi.org/10.1080/14693062.2020.1724070.
[43] Kim, D. and S. Song (2019), “The multifunctional benefits of green infrastructure in community development: An analytical review based on 447 cases”, Sustainability, Vol. 11/14, pp. 1-17, http://dx.doi.org/10.3390/su11143917.
[46] Koks, E. et al. (2019), “A global multi-hazard risk analysis of road and railway infrastructure assets”, Nature Communications, Vol. 10/1, p. 11, http://dx.doi.org/10.1038/s41467-019-10442-3.
[66] Krauß, W. and S. Bremer (2020), “The role of place-based narratives of change in climate risk governance”, Climate Risk Management, Vol. 28, pp. 1-7, https://doi.org/10.1016/j.crm.2020.100221.
[94] Kwon, Y., S. Son and K. Jang (2018), “Evaluation of incentive policies for electric vehicles: An experimental study on Jeju Island”, Transportation Research Part A: Policy and Practice, Vol. 116, pp. 404-412.
[134] Lorie, M. et al. (2020), “Modeling coastal flood risk and adaptation response under future climate conditions”, Climate Risk Management, Vol. 29, pp. 1-16, https://doi.org/10.1016/j.crm.2020.100233.
[50] Marí-Dell’Olmo, M. et al. (2019), “Social inequalities in the association between temperature and mortality in a South European context”, International Journal of Public Health, Vol. 64/1, pp. 27-37, http://dx.doi.org/10.1007/s00038-018-1094-6.
[24] Matsumoto, T. et al. (2019), “An integrated approach to the Paris climate Agreement: The role of regions and cities”, OECD Regional Development Working Papers, No. 2019/13, OECD Publishing, Paris, https://dx.doi.org/10.1787/96b5676d-en.
[45] Melvin, A. et al. (2017), “Climate change damages to Alaska public infrastructure and the economics of proactive adaptation”, Proceedings of the National Academy of Sciences, Vol. 114/2, pp. E122-E131, http://dx.doi.org/10.1073/pnas.1611056113.
[34] Mercure, J. et al. (2018), “Macroeconmic impact of stranded fossil fuel assets”, Nature Climate Change, http://dx.doi.org/10.1038/s41558-018-0182-1.
[39] Munich Re (n.d.), NatCatServices (database).
[51] Nakashima, D. et al. (2012), Weathering Uncertainty: Traditional Knowledge for Climate Change Assessment and Adaptation, UNESCO, Paris and UNU, Darwin.
[18] New Climate Economy (2019), Unlocking the Inclusive Growth Story of the 21st Century: Accelerating Climate Action in Urgent Times.
[70] OECD (2021), Toolkit for Water Policies and Governance: Converging Towards the OECD Council Recommendation on Water, OECD Publishing, Paris, https://doi.org/10.1787/ed1a7936-en.
[80] OECD (2020), Common Ground Between the Paris Agreement and the Sendai Framework: Climate Change Adaptation and Disaster Risk Reduction, OECD Publishing, Paris, https://dx.doi.org/10.1787/3edc8d09-en.
[61] OECD (2020), “COVID-19 and the low-carbon transition: Impacts and possible policy responses”, OECD Policy Responses to Coronavirus (COVID-19), OECD, Paris, http://www.oecd.org/coronavirus/policy-responses/covid-19-and-the-low-carbon-transition-impacts-and-possible-policy-responses-749738fc/.
[52] OECD (2020), Linking Indigenous Communities with Regional Development in Canada, OECD Rural Policy Reviews, OECD Publishing, Paris, https://dx.doi.org/10.1787/fa0f60c6-en.
[14] OECD (2020), Managing Environmental and Energy Transitions for Regions and Cities, OECD Publishing, Paris, https://doi.org/10.1787/f0c6621f-en.
[88] OECD (2020), OECD Regions and Cities at a Glance 2020, OECD Publishing, Paris, https://doi.org/10.1787/959d5ba0-en.
[1] OECD (2019), Accelerating Climate Action: Refocusing Policies through a Well-being Lens, OECD Publishing, Paris, https://dx.doi.org/10.1787/2f4c8c9a-en.
[121] OECD (2019), Enhancing Climate Change Mitigation through Agriculture, OECD Publishing, Paris, https://dx.doi.org/10.1787/e9a79226-en.
[31] OECD (2018), Cost-Benefit Analysis and the Environment: Further Developments and Policy Use, OECD Publishing, Paris, https://dx.doi.org/10.1787/9789264085169-en.
[23] OECD (2018), Effective Carbon Rates 2018: Pricing Carbon Emissions Through Taxes and Emissions Trading, OECD Publishing, Paris, https://dx.doi.org/10.1787/9789264305304-en.
[97] OECD (2018), Taxing Energy Use 2018: Companion to the Taxing Energy Use Database, OECD Publishing, Paris, https://dx.doi.org/10.1787/9789264289635-en.
[26] OECD (2017), Investing in Climate, Investing in Growth, OECD Publishing, Paris, https://dx.doi.org/10.1787/9789264273528-en.
[74] OECD (2003), Emerging Risks in the 21st Century: An Agenda for Action, OECD Publishing, Paris, https://dx.doi.org/10.1787/9789264101227-en.
[16] OECD (n.d.), Green Growth (database), OECD, Paris.
[40] OECD/EC (2020), Cities in the World: A New Perspective on Urbanisation, OECD Urban Studies, OECD Publishing, Paris, https://doi.org/10.1787/d0efcbda-en.
[96] OECD/ITF (2019), Tax Revenue Implications of Decarbonising Road Transport: Scenarios for Slovenia, OECD Publishing, Paris, https://dx.doi.org/10.1787/87b39a2f-en.
[36] Okazumi, T. and T. Nakasu (2015), “Lessons learned from two unprecedented disasters in 2011 – Great East Japan Earthquake and Tsunami in Japan and Chao Phraya River flood in Thailand”, International Journal of Disaster Risk Reduction, Vol. 13, pp. 200-206, https://doi.org/10.1016/j.ijdrr.2015.05.008.
[64] Pelling, M. (2010), Adaptation to Climate Change: From Resilience to Transformation, Taylor & Francis Group, https://www.taylorfrancis.com/books/mono/10.4324/9780203889046/adaptation-climate-change-mark-pelling.
[73] Pescaroli, G. et al. (2018), “Increasing resilience to cascading events: The M.OR.D.OR. scenario”, Safety Science, Vol. 110, pp. 131-140, https://doi.org/10.1016/j.ssci.2017.12.012.
[78] Renn, O. (2016), “Systemic risks: The new kid on the block”, Environment: Science and Policy for Sustainable Development, Vol. 58/2, pp. 26-36, http://dx.doi.org/10.1080/00139157.2016.1134019.
[6] Rohr, J. et al. (2019), “Emerging human infectious diseases and the links to global food production”, Nature Sustainability, Vol. 2, pp. 445-456, http://dx.doi.org/10.1038/s41893-019-0293-3.
[103] Roy, R. and N. Braathen (2017), “The Rising Cost of Ambient Air Pollution thus far in the 21st Century: Results from the BRIICS and the OECD Countries”, OECD Environment Working Papers, No. 124, OECD Publishing, Paris, https://dx.doi.org/10.1787/d1b2b844-en.
[118] Rydge, J., R. Martin and A. Valero (2018), “Sustainable growth in the UK: Seizing the opportunities from technological change and the transition to a low-carbon economy”, Grantham Research Institute on Climate Change and the Environment, London.
[32] Sanderson, B. and B. O’Neill (2020), “Assessing the costs of historical inaction on climate change”, Scientific Reports, Vol. 10/1, pp. 1-12, http://dx.doi.org/10.1038/s41598-020-66275-4.
[128] Schoof, J. and S. Robeson (2016), “Projecting changes in regional temperature and precipitation extremes in the United States”, Weather and Climate Extremes, Vol. 11, pp. 28-40, https://doi.org/10.1016/j.wace.2015.09.004.
[108] Schucht, S. et al. (2015), “Moving towards ambitious climate policies: Monetised health benefits from improved air quality could offset mitigation costs in Europe”, Environmental Science & Policy, Vol. 50, pp. 252-269, https://doi.org/10.1016/j.envsci.2015.03.001.
[72] Shepherd, T. and A. Sobel (2020), “Localness in climate change”, Comparative Studies of South Asia, Africa and the Middle East, Vol. 40/1, pp. 7-16, http://dx.doi.org/10.1215/1089201X-8185983.
[135] Soto-Montes-de-Oca, G. and M. Alfie-Cohen (2019), “Impact of climate change in Mexican peri-urban areas with risk of drought”, Journal of Arid Environments, Vol. 162, pp. 74-88, https://doi.org/10.1016/j.jaridenv.2018.10.006.
[7] Steffen, W. et al. (2018), “Trajectories of the Earth System in the anthropocene”, PNAS, Vol. 115/33, pp. 8252-8259, http://dx.doi.org/10.1073/pnas.1810141115/-/DCSupplemental.
[77] Suo, W., J. Zhang and X. Sun (2019), “Risk assessment of critical infrastructures in a complex interdependent scenario: A four-stage hybrid decision support approach”, Safety Science, Vol. 120, pp. 692-705, https://doi.org/10.1016/j.ssci.2019.07.043.
[56] Sussams, L., W. Sheate and R. Eales (2015), “Green infrastructure as a climate change adaptation policy intervention: Muddying the waters or clearing a path to a more secure future?”, Journal of Environmental Management, Vol. 147, pp. 184-193, https://doi.org/10.1016/j.jenvman.2014.09.003.
[67] Taylor, F. et al. (2020), “Messy maps: Qualitative GIS representations of resilience”, Landscape and Urban Planning, Vol. 198, pp. 1-11, https://doi.org/10.1016/j.landurbplan.2020.103771.
[130] Tezuka, S. et al. (2014), “Estimation of the effects of climate change on flood-triggered economic losses in Japan”, International Journal of Disaster Risk Reduction, Vol. 9, pp. 58-67, https://doi.org/10.1016/j.ijdrr.2014.03.004.
[81] UNDP (2020), COVID-19 and Human Development: Assessing the Crisis, Envisioning the Recovery, United Nations Development Programme, http://hdr.undp.org/sites/default/files/covid-19_and_human_development_0.pdf.
[9] UNEP (2020), Emissions Gap Report 2020, United Nations Environment Programme.
[10] UNEP (2019), Emissions Gap Report 2019, United Nations Environment Programme, https://www.unenvironment.org/resources/emissions-gap-report-2019 (accessed on 19 July 2020).
[119] Unsworth, S. et al. (2020), “Seizing sustainable growth opportunities from zero emissions passenger vehicles in the UK”, Grantham Research Institute on Climate Change and the Environment, London, Working Paper.
[35] Ürge-Vorsatz, D., B. Boza-Kiss and S. Chatterjee (2019), “What policies can prepare cities and regions for the transition to a climate-neutral economy?”, Background paper for an OECD/EC Workshop on 17 May 2019 within the workshop series “Managing environmental and energy transitions for regions and cities".
[57] Venkataramanan, V. et al. (2019), “A systematic review of the human health and social well-being outcomes of green infrastructure for stormwater and flood management”, Journal of Environmental Management, Vol. 246, pp. 868-880, https://doi.org/10.1016/j.jenvman.2019.05.028.
[104] WHO (2018), Air Pollution and Child Health: Prescribing Clean Air. Summary, World Health Organization, Geneva, http://apps.who.int/bookorders.
[110] WHO/JRC (2011), “Burden of disease from environmental noise: Quantification of healthy life years lost in Europe”, World Health Organization.
[49] Winsemius, H. et al. (2018), “Disaster risk, climate change, and poverty: Assessing the global exposure of poor people to floods and droughts”, Environment and Development Economics, Vol. 23/3, pp. 328-348, http://dx.doi.org/10.1017/S1355770X17000444.
[30] Wolkinger, B. et al. (2018), “Evaluating health co-benefits of climate change mitigation in urban mobility”, International Journal of Environmental Research and Public Health, Vol. 15/5, p. 880, http://dx.doi.org/10.3390/ijerph15050880.
[91] WRI (n.d.), Global Power Plant Database, World Resources Institute, https://datasets.wri.org/dataset/globalpowerplantdatabase.
[29] Xie, Y. et al. (2018), “Co-benefits of climate mitigation on air quality and human health in Asian countries”, Environment International, Vol. 119, pp. 309-318.
[42] Zhang, Y. et al. (2018), “The MJA–Lancet Countdown on health and climate change: Australian policy inaction threatens lives”, Medical Journal of Australia, Vol. 209/11, pp. 474e1-474e21, http://dx.doi.org/10.5694/mja18.00789.
[75] Zscheischler, J. et al. (2018), “Future climate risk from compound events”, Nature Climate Change, Vol. 8/6, pp. 469-477, http://dx.doi.org/10.1038/s41558-018-0156-3.