This chapter discusses the untapped potential of systems innovation and how incentives for cleaner vehicles’ uptake and carbon prices could be implemented to be more effective and to contribute to wider systems redesign. The chapter also discusses how measurement frameworks overestimate the mitigation potential of policies, biasing decisions towards improved vehicle performance.
Transport Strategies for Net-Zero Systems by Design
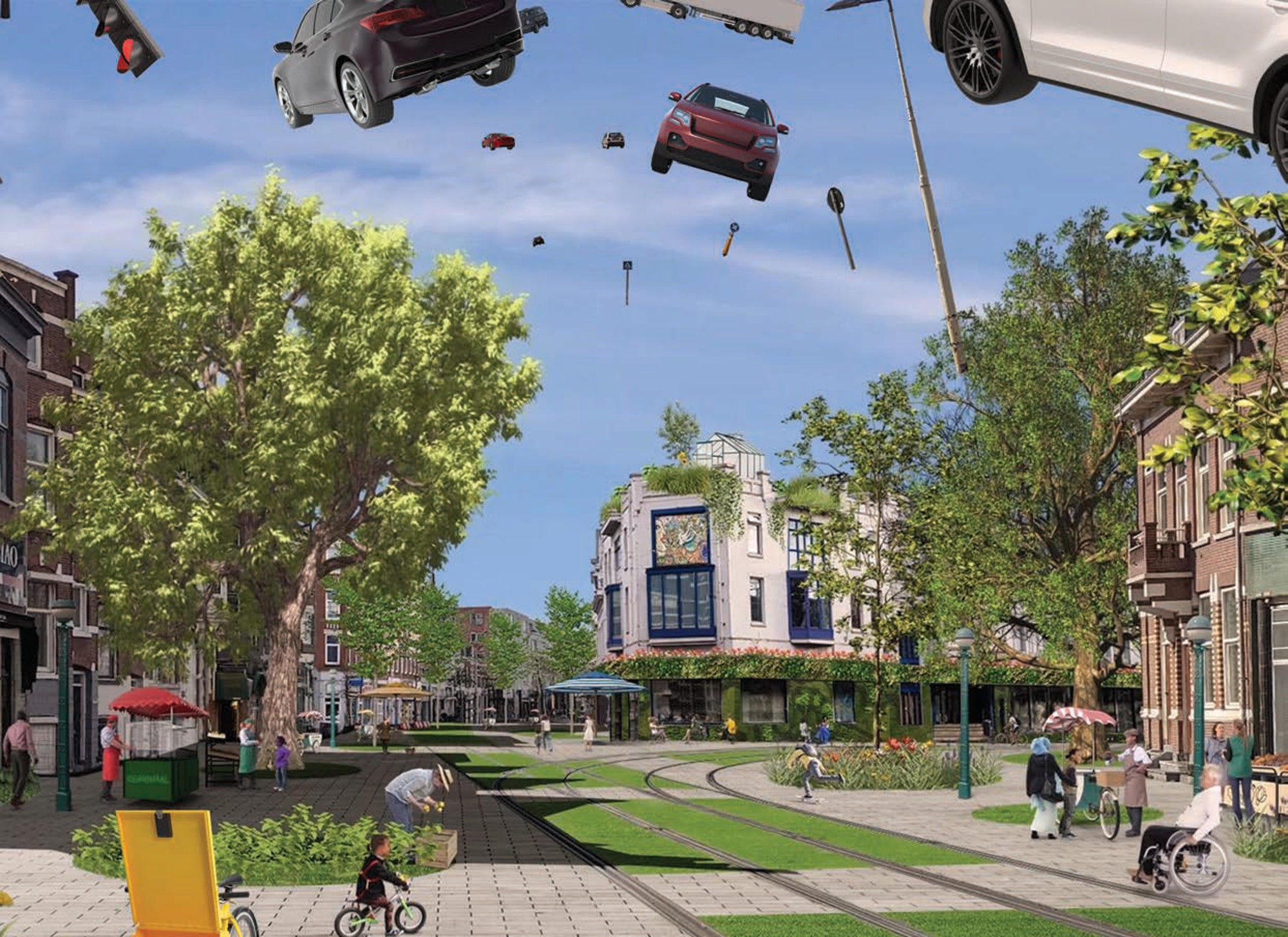
6. Innovation and carbon prices for systems redesign
Abstract
Policies fostering innovation and technological change such as incentives for cleaner vehicles’ uptake, as well as market-based instruments such as carbon prices play a central role in current climate strategies. While fundamental to achieve mitigation goals, the potential of these instruments to mitigate emissions is not always fulfilled, synergies with other well-being outcomes are often missed, while important trade-offs are also created.
The effectiveness (both for decarbonisation and other benefits) and the feasibility of these instruments could be much larger if these were implemented as part of a wider strategy to foster systems innovation. Failing to do so can instead, importantly lock-in emissions, either by reinforcing the dynamics described in chapters 3-5 (thus off-setting to a great extent any progress in reducing emissions per vehicles); or by failing to create the right conditions for widespread change towards more sustainable choices (often also translating into roll-back of policies like carbon pricing).
This chapter discusses key considerations to avoid that incentives and enabling infrastructure to accelerate the uptake of better vehicle technologies foster car-dependency, as well as the ways in which systemic redesign could help increase the effectiveness and feasibility of carbon pricing . A final section argues that overall, better methodologies for measuring and comparing the impacts of different policies is needed to steer decisions towards untapping the synergies between innovating at the parts and the systems level, which is crucial given the scale of the climate challenge.
6.1. The untapped potential of systems innovation
As discussed in chapter 2, Systems innovation is innovation aimed at transforming the systems’ functioning (Systems Innovation, 2020[1]), and while in some cases requires advanced technologies, it is also often about changes that are not technological. Superblocks in Barcelona, described in Chapter 3, are an example of low-tech systems innovation. Superblocks innovate in the way in which public space is allocated and designed, thus modifying the systems’ structure and significantly affecting people’s transport modes choices.
Advanced technologies open up enormous opportunities for systems innovation, and many synergies can be created between innovating at the parts and the systems level. For example, GPS technologies and apps today allow people to share vehicles (e.g. bikes) and combine transport modes in ways that were unimaginable just a few decades ago. However, without embedding GPS technology and apps in an attempt to redesign transport and urban systems to shift them away from car-dependency, these technologies have so far led more to the uptake of ride-hailing (e.g. Uber-like non-shared services); without really helping to overcome the challenges to decarbonise the sector (and in some cases making it more challenging). These technologies could instead allow the shift from a system which functioning requires each person to own a car, and where cars have an utilisation rate of 5% in average (Shoup (2005[2]) accessed from Franco (2020[3])), to systems in which a multiplicity of transport modes are available for people to choose and combine according to their needs and have access to cars as a service. In such systems, much smaller car fleets would be needed, which would make achieving high shares of electric vehicles in the short-term more plausible and would entail less potential trade-offs, as less minerals (and thus mining) will be required. In addition a system in which a high number of vehicles are managed as part of a same fleet and where vehicles utilisation rates are higher, can help accelerate vehicle turn over and thus the uptake of cleaner technologies, while also facilitating the uptake of new technologies for better managing the demand for electricity (e.g. vehicle-to-grid (V2G) technologies (Gschwendtner, 2021[4]); also reducing potential pressure on the energy sector. At the same time, such a system can also increase the feasibility and effectiveness of market-based instruments such as carbon pricing by creating better conditions for people to make more sustainable choices; thus reducing the potential distributional negative outcomes and raising acceptability.
Unfortunately, most of these potential synergies remain untapped, and for the contrary, often carbon strategies (following a decoupling logic- see Chapter 2) focus on decarbonising vehicles and correcting pricing, while leaving car-dependency practically intact. The rest of this section discusses policies and charging infrastructure for cleaner vehicles, as well as carbon pricing in the context of system’s redesign.
6.1.1. Innovation in parts that advances systems redesign: incentives and charging infrastructure for cleaner vehicles
Both fuel economy standards (targeting vehicle supply) and tax incentives to purchase cleaner vehicles (targeting vehicle demand and influencing supply indirectly) are important instruments to improve the fuel efficiency and emissions performance of the vehicle fleet (Wappelhorst, Mock and Yang, 2018[5]). Vehicle purchase taxes and circulation taxes (one-off or annual charges) are the most common tax incentive mechanisms to encourage the purchase of low-carbon (including electric) vehicles. In some countries, revenues from the purchase taxes of more polluting vehicles are used to grant rebates/subsidies to cleaner vehicles (often referred to as “fee-bate programmes”). In addition, due to price differentials between combustion and electric vehicles,1 additional incentives may be needed to accelerate their uptake2, and a number of countries have increasingly made use of these incentives.3
Norway is a front-runner in moving forward in electrifying its vehicle fleet and uses a wide range of tax and non-tax incentives for reducing the generalised cost of driving. In 2017, electric vehicles accounted for 39% of all new car sales in Norway, turning the country into the most advanced market for EVs worldwide (IEA, 2020[6]), and Oslo into the “electric vehicle capital” (Holtsmark and Skonhoft, 2014[7]). Between 1992 and 1997, the Norwegian government introduced a registration tax exemption for EVs, free public parking, a reduction in the annual circulation tax and the company car tax, and an exemption from the road toll and ferry charge. A value-added tax exemption for battery electric vehicles also entered into force in 2001. Additionally, since 2005, EVs are allowed to use lanes that are reserved for buses and taxis (Lindberg and Fridstrøm, 2015[8]), and can change their battery for free (Holtsmark and Skonhoft, 2014[7]). A significant part of the combustion engine vehicle tax revenues has been used to support the development of charging infrastructure (IEA, 2020[6]).
The Norwegian case provides two key lessons. First, while packages of incentives can certainly help, mainstreaming incentives into the wider fiscal system is key. The fiscal system in Norway includes a number of CO2-based related taxes, equivalent to paying a carbon tax higher than EUR 1 250 per tonne (Fridstrøm, 2020[9]). This shows the potential of embedding EV incentives into wider fiscal reforms.
Second, special attention needs to be given so that incentives included in packages do not reinforce car dependency. While the share of EVs has importantly increased, evidence suggests that car travel and ownership in Norway is also being incentivised as a result of the incentive packages (Holtsmark and Skonhoft, 2014[7]). Specifically, allowing EVs to use bus lanes has resulted in more bus congestion, potentially reducing their attractiveness vis-à-vis cars, and thus exacerbating rather than helping reverse the erosion of sustainable modes. Exempting EVs from parking fees also locks-in space for cars (instead of other modes and uses) and incentivises their use over active or shared modes. While the replacement of combustion cars with EVs can reduce tail-pipe CO2 emissions, air pollution and noise,4 non-exhaust emissions, and other social costs (e.g. road fatalities), inequality of access remains, and may be exacerbated. A bigger EV fleet also exacerbates trade-offs with other environmental impacts, e.g. related to minerals extractions for batteries (Holtsmark and Skonhoft, 2014[7]) (see Chapter 2). EV incentives designed, instead, from a systemic approach, i.e. with careful attention of not exacerbating car dependency, could become more effective in reducing emissions while simultaneously achieve other well-being goals.
Importantly, and adding to the discussion on measurement biases at the end of this chapter, evaluation of incentives for the purchase of cleaner vehicles needs to be compared to other policy and investment options, such as incentives for modal shifts towards active and shared modes, or policies and infrastructure investments fostering the creation of proximity to avoid trips as much as possible. Instead, estimated CO2 emissions reductions from the introduction of EVs are most often compared to emissions in a scenario in which vehicles remain combustion-based. The measurement reflects the implicit assumption that the number of vehicles is a given (rather than a result of the system’s design, as explained in Section 3.1).
Charging infrastructure is also fundamental for the uptake of EVs (Falchetta and Noussan, 2021[10]), and its deployment needs to be significantly accelerated to meet stringent international mitigation targets. The stock of charging infrastructure increased by 40% between 2018 and 2019 (IEA, 2020[6]), with France, Germany, Italy, the Netherlands, Norway, Sweden and the United Kingdom being the countries with the highest installed capacity as of November 2020 (Falchetta and Noussan, 2021[10]). The IEA estimates however that, for instance, the number of charging facilities needs to almost double (to around 20 million) to reach emissions goals in the Sustainable Development Scenario5 (IEA, 2020[6]).
Policies to accelerate the transition towards car independent systems could reduce charging facility needs, facilitating reaching electrification targets and reducing the costs to do so. For instance, San Francisco aims for a 100% EV fleet by 2030, and a study of infrastructure needs to deliver such goal finds that public charging infrastructure needs could be reduced by 45% if 80% of trips are “non-automobile” (walking, biking, transit); another goal of San Francisco’s strategy (SFMTA, 2017[11]) (Figure 6.1). As a result, 2 900 instead of 5 100 public charging stations would be needed by 2030, reducing the required growth rate of installation from 18% to 12%, thus facilitating the strategy’s implementation (Hsu, Slowik and Lutsey, 2020[12]).
Figure 6.1. San Francisco modal shift goal by 2030
In turn, the deployment of charging infrastructure could foster system redesign. Mobility hubs and charging stations with services for different transport modes can facilitate modal shifts from private cars and reduce car dependency (Transport & Environment, 2020[13]). Mobility hubs are locations in which sustainable transport modes such as (e-)bikes, cargo or children’s bikes, and e-scooters could be made available (Transport & Environment, 2020[13]). Charging stations with services for different users can, for instance, provide slow chargers for park & ride (i.e. people leaving their car parked to take public transportation, for example at the outskirts of cities), as well as fast charging for long-distance trips, taxis, ride-sharing services and commercial delivery vans (Transport & Environment, 2020[13]). Cities could develop joint energy, transport and telecom plans to find the best locations for developing new hubs and for assessing the needs in terms of charging facilities for existing hubs (Transport & Environment, 2020[13]).
Other actions to facilitate the deployment of charging infrastructure include incorporating charging points’ standards into real estate development (e.g. introducing targets for the shares of parking space with charging points) and adding charging stations to existing parking spaces. To reduce on-road parking and traffic and liberate urban space for other uses (e.g. electric micro-mobility), such actions need to go hand‑in-hand with the revision of parking space regulation and the removal of minimum parking requirements where these are still in place (as discussed in Chapters 3 and 4). To account for equity considerations, Transport & Environment (2020[13]) recommends targets for the development of charging facilities and shared EV services in low-income areas.
6.1.2. Carbon prices and systems redesign
The most widely used instrument to price carbon emissions in the road sector across OECD countries are fuel excise taxes (OECD, 2019[14]). Fuel excise taxes are considered to be the most efficient policy instrument for promoting behavioural change to reduce carbon emissions via reduced driving, a shift towards fuel-efficient vehicles and the use of more sustainable transport modes (van Dender, 2019[15]).A study in Canada finds as well that carbon pricing has contributed to reduced driving by containing urban sprawl, one of the three dynamics described in this report. The authors find that, on average, a 1% increase in gas prices led to a 0.32% increase in the population in city centres and a 0.60% decrease in low-density housing (Tanguay and Gingras, 2012[16]).
Evidence suggests, however, that carbon pricing alone will not translate into emission reductions at the pace and scale needed, even at high prices (Tvinnereim and Mehling, 2018[17]; Rosenbloom et al., 2020[18]). Rosenbloom et al. (2020[18]) analyse carbon pricing from a systemic perspective and suggest that a complex problem like climate change cannot be solved solely through market instruments, and that climate strategies focused primarily on carbon pricing will not result in the transformative change needed to reach net-zero goals on time. The authors call for policies triggering changes across new vehicle technologies, infrastructure, business models, regulation and city planning (Rosenbloom et al., 2020[18]).
In addition, setting carbon prices has been politically challenging, with public resistance resulting in policy roll-back or stagnation in a number of countries (Douenne and Fabre, 2020[19]) and almost 40% of emissions in road transport priced below central estimates of the carbon price that would be needed by 2030 to decarbonise the economy by mid-century (e.g. EUR 120 by 2030) (OECD, 2021[20])6. The impact of fuel prices on people’s choice is also low when alternatives to car driving are not available or limited, as illustrated in Figure 6.2 (Geman, 2019[21]).
Figure 6.2. Gasoline prices and vehicle miles travelled in the United States
Nonetheless, combining carbon prices with policies contributing to car independence can significantly increase their mitigation potential (Rosenbloom et al., 2020[18]; Kaufman et al., 2020[22]), as well as their acceptance. Behavioural change would be easier, and possible in the first place, if alternatives to car use were available and convenient. The revenue raised by carbon pricing could also help enhance public support and address equity concerns; for instance, through supporting sustainable mobility projects. Policies are also likely to have a higher acceptability if they are framed as part of a larger package with environmental and/or social benefits (rather than being perceived as a revenue collection mechanism), and if communication efforts shed light on policy efforts to, in parallel to pricing, improve access to alternative transport modes and tackle equity considerations (ITF, 2017[23]; 2018[24]).
A number of studies have shown, for instance, that public transport infrastructure can significantly change the elasticity of travel and energy demand (and related greenhouse gas emissions) and increase the responsiveness to carbon pricing in the transport sector. Avner, Rentschler and Hallegatte (2014[25]) estimate that price elasticity of CO2 emissions from private car travel is twice as high in a region like Paris, with a dense public transport network, than it is in areas without such a dense network. The authors find that the absence of dense public transport systems increases transport emissions while importantly limiting the territory’s capacity to mitigate. Similarly, Gillingham and Munk-Nielsen (2019[26]) find that in Denmark, the price elasticity of driving is higher among people that live centrally in urban areas and have the shortest commutes. They highlight that, in contexts where access to public transport is limited (e.g. many cities in the United States), price elasticities are low since there is no easy way to shift away from car use (e.g. due to the absence of commuting trains). Mattioli, Wadud and Lucas (2018[27]) show that in the United Kingdom, low-income households with car-related economic stress (e.g. transport representing a high share of their budget) show the lowest price elasticities to fuel price increases, likely due to a lack of alternatives. The authors develop a vulnerability index to fuel price increases by combining exposure (cost burden of motor fuel), sensitivity (income level) and adaptive capacity (access to alternative transport infrastructure) (Mattioli et al., 2019[28]). Analysis based on this type of index can help identify vulnerable households, infrastructure gaps and necessary compensatory measures, as well as potential acceptability issues related to carbon pricing increases.
6.2. Better measurement for striking a balance between innovation in parts and systems innovation
While improving vehicles’ emission performance is important to reduce emissions in the sector, data suggests that efforts to electrify vehicles have been ineffective in reducing emissions at the pace needed, and the potential of such efforts has systematically been overestimated, biasing decision-making. For example, Lamb et al. (2021[29]) find that emission reductions from electrification efforts in the transport sector have largely been offset by emission increase due to growing traffic.
As discussed in Litman (2017[30]), there are important omissions, of both negative and positive impacts, in the way emissions reduction options are evaluated. These omissions can reinforce deeply engrained ideas such as technological optimism and fossil fuel solutionism7 (see Chapter 2), which in turn bias decision-making towards climate strategies focused on a decoupling logic, i.e. on improving vehicles rather than on focusing on how to reduce traffic volumes (Litman, 2017[30]). The rest of this section discusses two measurement biases that influence policy decisions: the failure to reflect rebound effects from improved vehicle efficiency, and the gap between laboratory and real-world emissions.
Research by Litman (2017[30]) suggests that rebound effects from improved vehicle efficiency are seldom, if at all, included in climate strategies and programmes assessments. This leads to an overestimation of the mitigation potential of improved vehicle efficiency, while also underestimating the importance of systemic policies. Long-term rebound effects range between 15% and 30% (Litman, 2017[30]). For example, a 20% rebound effect means that, everything else equal, a 50% increase in fuel economy will increase travel by 10%; thus energy savings will only be 40% (Litman, 2017[30]). Due to the rebound effect, policies improving vehicle efficiency can also cause other negative impacts such as congestion, accidents, and inefficient use of public space, rarely accounted for in decision-making processes (Litman, 2017[30]). As discussed in Anable (2008[31]), “the rebound effect is not inevitable”, but minimising it requires policies that can restrain (and actually reverse) demand increases (such as those discussed in Chapters 3-5).
The growing gap between laboratory and real-world emissions is another barrier for informed policy decisions, which also translates in overestimating the potential of policies focused on improving vehicle technologies. The gap between emission testing and real-life emissions has widened significantly over the years, implying that vehicles are in reality less efficient than what emission testing shows. For example, in Europe, research suggests that the gap has increased from 9% in 2001 to 28% in 2012 and 42% in 2015 (Transport & Environment, 2016[32]), and that, in contrast to what emission tests show, on-road efficiency in Europe stalled between 2012 and 2016 (Transport & Environment, 2016[32]). Transport & Environment (2016[32]) finds that the gap between laboratory and on‑road testing could translate into an extra 1.5 billion tonnes of CO2 emissions in Europe by 2030.
In addition to misinforming policy decisions, the gap between emission testing and real emissions affect car drivers. In Europe, car drivers spend annually EUR 549 more than the cost expected when looking at the fuel economy levels of their vehicles (Transport & Environment, 2016[32]). By 2030, this could add up to a cumulative excess expenditure by European drivers equal to EUR 1 trillion; and an import of around 6 billion extra barrels of oil at the EU level (Transport & Environment, 2016[32]).
The gap is particularly wide for hybrid and plug-in hybrid vehicles (PHEVs) (Transport & Environment, 2016[32]). Studies show that PHEVs’ on-road emission levels are significantly higher than the level of emissions that are registered for these vehicles, and higher than the thresholds (e.g. 50 kg CO2/km in Europe) that make a vehicle eligible for preferential treatment. For instance, tests on the three most popular and performing PHEVs in Europe reveal that they can emit three to four times more than official levels (Bannion, 2020[33]; Plötz et al., 2020[34]). The gap can be up to 8 times higher for less performant vehicles, and up to 12 times in the case of vehicles designed for geo-fencing, i.e. when the engine is used to recharge the battery. This could for instance be problematic in the case of low-emission zones, as there is an incentive to drive in this mode before entering the LEZ area (Berman, 2020[35]).
Ignoring the wide gap in the case of hybrids and PHEVs has mislead policies in a number of ways. For example, hybrid and PHEVs have become eligible for preferential treatment, often being eligible for tax reductions and other incentives such as super credits for fuel economy standards in Europe. They are also exempted from bans and/or included in EV mandates, which has contributed to boosting their sales (Bannion, 2020[33]), locking in emissions, and reducing climate policy effectiveness (Brand et al., 2020[36]).
Expanding the shift (having already taken place in Europe and Japan) from tests based on theoretical driving (the New European Driving Cycle) to tests based on real driving (the Worldwide Harmonised Light Vehicle Test Procedure) (WLTPfacts.eu, n.d.[37]) is an important step to reducing the widening gap between laboratory and real emissions. The shift towards the Worldwide Harmonised Light Vehicle Test Procedure will influence a number of key policies (e.g. fuel economy and CO2 emission standards, CO2-based taxation and labelling) by allowing targets and criteria that better reflect real emissions levels (for more see WLTPfacts.eu (n.d.[37])).
Beyond the type of test, the extensive and increased flexibilities (e.g. wheel and tyre specification, tyre pressure, running temperature, among others) permitted during testing procedures are another key factor underlying an increasing gap. Flexibility has allowed manufacturers to optimise certain testing conditions to achieve lower fuel consumption and CO2 emissions (European Environment Agency, 2016[38]). For example, in Europe, while the gap between test and on-road emissions would be between 19% and 28% without flexibilities, with flexibilities it reaches on average 38%, and even up to 50% (Transport & Environment, 2016[32]).
To improve measurement, Transport & Environment (2016[32]) calls for the implementation of road testing (with the use of portable emissions monitoring systems) and for regulations to limit discrepancies between laboratory and on-road tests to 10%. On-road test checks will importantly reduce the incentives for relying on flexibilities and can steer innovation towards vehicles that perform well on-road rather than in the laboratory (Transport & Environment, 2016[32]). Along these lines, the 2020-21 European CO2 and fuel economy standards already incorporated the regular monitoring of on-road emissions data through on‑board devices (European Commission, 2020[39]).8 Other ways forward include: strengthening testing frameworks, bringing attention to good practice, advocating for the establishment of surveillance authorities, introducing independent conformity checks and strengthening testing frameworks, and random testing and intensive audits on car manufacturers’ own tests (as already done by the United States’ Environmental Protection Agency) (Transport & Environment, 2016[32]).
As a way forward, a better reflection of the rebound effect and real-world emissions are key for more informed policy decisions. Better measurement could help policy makers shift away from technological optimism and fossil fuel solutionism, and better assess the importance of putting systems redesign efforts at the centre of climate strategies.
References
[31] Anable, J. (2008), The Cost-effectiveness of Carbon Abatement in the Transport Sector, Campaign for Better Transport, London, https://bettertransport.org.uk/sites/default/files/research-files/Carbon_abatement_research.pdf.
[25] Avner, P., J. Rentschler and S. Hallegatte (2014), “Carbon price efficiency: Lock-in and path dependence in urban forms and transport infrastructure”, Policy Research Working Paper, World Bank, Washington, DC, https://doi.org/10.1596/1813-9450-6941 (accessed on 29 March 2021).
[33] Bannion, E. (2020), “Plug-in hybrids: Is Europe heading for a new dieselgate?”, European Federation for Transport and Environment, Brussels, https://www.transportenvironment.org/publications/plug-hybrids-europe-heading-new-dieselgate (accessed on 10 December 2020).
[35] Berman, B. (2020), “Should plug-in hybrids be forced into EV mode in cities? Fiat tests the tech”, Electrek, https://electrek.co/2020/06/03/should-plug-in-hybrids-be-forced-into-ev-mode-in-cities-fiat-tests-the-tech (accessed on 28 July 2021).
[36] Brand, C. et al. (2020), “Road to zero or road to nowhere? Disrupting transport and energy in a zero carbon world”, Energy Policy, Vol. 139, p. 111334, http://dx.doi.org/10.1016/j.enpol.2020.111334.
[19] Douenne, T. and A. Fabre (2020), Yellow Vests, Carbon Tax Aversion, and Biased Belifes, Paris School of Economics, Paris, https://www.parisschoolofeconomics.eu/docs/douenne-thomas/job-market-paper--yellow-vests,-carbon-tax-aversion,-and-biased-beliefs.pdf (accessed on 26 March 2021).
[39] European Commission (2020), CO₂ emission performance standards for cars and vans | Climate Action, https://ec.europa.eu/clima/policies/transport/vehicles/regulation_en (accessed on 5 October 2021).
[38] European Environment Agency (2016), “Explaining vehicle emissions: Why do laboratory and road measurements differ?”, European Environment Agency, https://www.eea.europa.eu/highlights/explaining-vehicle-emissions (accessed on 26 March 2021).
[10] Falchetta, G. and M. Noussan (2021), “Electric vehicle charging network in Europe: An accessibility and deployment trends analysis”, Transportation Research Part D: Transport and Environment, Vol. 94, p. 102813, http://dx.doi.org/10.1016/J.TRD.2021.102813.
[3] Franco, S. (2020), “Parking prices and availability, mode choice and urban form”, International Transport Forum Discussion Papers, No. 2020/03, OECD Publishing, Paris, https://doi.org/10.1787/04ae37c3-en.
[9] Fridstrøm, L. (2020), The Carbon Price Implicit in the Norwegian Road, Fuel and Vehicle Taxation System, Norwegian Institute of Transport, Oslo, https://trid.trb.org/view/1752155 (accessed on 26 March 2021).
[21] Geman, B. (2019), “Transportation emissions are a tough nut to crack”, Axios, https://www.axios.com/carbon-pricing-difficult-to-reduce-transportation-emissions-b6cf8a74-6cfe-47ca-9f6e-c5b4d266d96c.html (accessed on 30 August 2021).
[26] Gillingham, K. and A. Munk-Nielsen (2019), “A tale of two tails: Commuting and the fuel price response in driving”, Journal of Urban Economics, Vol. 109, pp. 27-40, http://dx.doi.org/10.1016/j.jue.2018.09.007.
[4] Gschwendtner, C. (2021), Vehicle-to-Grid (V2G) and car sharing: Joining forces to decarbonize electricity and transport?, https://blogs.ethz.ch/energy/v2g-and-car-sharing-joining-forces/ (accessed on 10 August 2021).
[7] Holtsmark, B. and A. Skonhoft (2014), “The Norwegian support and subsidy policy of electric cars: Should it be adopted by other countries?”, Environmental Science & Policy, Vol. 42, pp. 160-168, http://dx.doi.org/10.1016/J.ENVSCI.2014.06.006.
[12] Hsu, C., P. Slowik and N. Lutsey (2020), City Charging Infrastructure Needs to Reach 100% Electric Vehicles: The Case of San Francisco, International Council on Clean Transportation, Washington, DC, https://theicct.org/publications/sf-ev-charging-infra-oct2020 (accessed on 2 December 2020).
[6] IEA (2020), Global EV Outlook 2020: Entering the Decade of Electric Drive?, OECD Publishing, Paris, https://dx.doi.org/10.1787/d394399e-en.
[24] ITF (2018), “Strategies for mitigating air pollution in Mexico City”, International Transport Forum Policy Papers, No. 30, OECD Publishing, Paris, https://doi.org/10.1787/bb049481-en.
[23] ITF (2017), Income Inequality, Social Inclusion and Mobility, ITF Roundtable Reports, No. 164, OECD Publishing, Paris, https://doi.org/10.1787/g2g7ae77-en.
[22] Kaufman, N. et al. (2020), “A near-term to net zero alternative to the social cost of carbon for setting carbon prices”, Nature Climate Change, Vol. 10/11, pp. 1010-1014, http://dx.doi.org/10.1038/s41558-020-0880-3.
[40] Lamb, W. et al. (2020), “Discourses of climate delay”, Global Sustainability, Vol. 3, http://dx.doi.org/10.1017/SUS.2020.13.
[29] Lamb, W. et al. (2021), “A review of trends and drivers of greenhouse gas emissions by sector from 1990 to 2018”, Environmental Research Letters, Vol. 16, http://dx.doi.org/10.1088/1748-9326/abee4e.
[8] Lindberg, G. and L. Fridstrøm (2015), “Policy strategies for vehicle electrification”, International Transport Forum Discussion Papers, No. 2015/16, OECD Publishing, Paris, https://doi.org/10.1787/5jrvzrldjs8n-en.
[30] Litman, T. (2017), Smart Transportation Emission Reduction Strategies, Victoria Transport Policy Institute, Victoria, British Columbia, https://www.vtpi.org/ster.pdf.
[28] Mattioli, G. et al. (2019), “Vulnerability to motor fuel price increases: Socio-spatial patterns in England”, Journal of Transport Geography, Vol. 78, pp. 98-114, http://dx.doi.org/10.1016/j.jtrangeo.2019.05.009.
[27] Mattioli, G., Z. Wadud and K. Lucas (2018), “Vulnerability to fuel price increases in the UK: A household level analysis”, Transportation Research Part A: Policy and Practice, Vol. 113, pp. 227-242, http://dx.doi.org/10.1016/j.tra.2018.04.002.
[20] OECD (2021), Effective Carbon Rates 2021: Pricing Carbon Emissions through Taxes and Emissions Trading, OECD Publishing, Paris, https://dx.doi.org/10.1787/0e8e24f5-en.
[14] OECD (2019), Taxing Energy Use 2019: Using Taxes for Climate Action, OECD Publishing, Paris, https://dx.doi.org/10.1787/058ca239-en.
[34] Plötz, P. et al. (2020), Real-World Usage of Plug-In Hybrid Electric Vehicles Fuel Consumption, Electric Driving, and CO2 Emissions, International Council on Clean Transportation, https://theicct.org/publications/phev-real-world-usage-sept2020 (accessed on 10 December 2020).
[18] Rosenbloom, D. et al. (2020), “Opinion: Why carbon pricing is not sufficient to mitigate climate change – and how “sustainability transition policy” can help”, Proceedings of the National Academy of Sciences, Vol. 117/16, pp. 8664-8668, http://dx.doi.org/10.1073/pnas.2004093117.
[11] SFMTA (2017), 2017 San Francisco Transportation Sector Climate Action Strategy, San Francisco Municipal Transportation Agency, San Francisco, CA, https://www.sfmta.com/sites/default/files/reports-and-documents/2017/12/12-5-17_item_15_transportation_sector_climate_action_strategy.pdf.
[2] Shoup, D. (2005), “The high cost of free parking”, in The Twenty-First Century Parking Problem, https://www.researchgate.net/publication/264967305_The_high_cost_of_free_parking (accessed on 13 August 2021).
[1] Systems Innovation (2020), Introduction to Systems Innovation, Systems Innovation, https://www.systemsinnovation.io/post/systems-innovation-guide (accessed on 29 March 2021).
[16] Tanguay, G. and I. Gingras (2012), “Gas price variations and urban sprawl: An empirical analysis of the twelve largest Canadian metropolitan areas”, Environment and Planning A, Vol. 44, pp. 1728-1743, http://dx.doi.org/10.1068/a44259.
[13] Transport & Environment (2020), Recharge EU: How many charge points will EU countries need by 2030, European Federation for Transport and Environment, Brussels, https://www.transportenvironment.org/discover/recharge-eu-how-many-charge-points-will-eu-countries-need-2030/ (accessed on 2 December 2020).
[32] Transport & Environment (2016), Mind the Gap 2016, European Federation for Transport and Environment, Brussels, https://www.transportenvironment.org/publications/mind-gap-2016-report (accessed on 26 March 2021).
[17] Tvinnereim, E. and M. Mehling (2018), “Carbon pricing and deep decarbonisation”, Energy Policy, Vol. 121, pp. 185-189, http://dx.doi.org/10.1016/j.enpol.2018.06.020.
[15] van Dender, K. (2019), “Taxing vehicles, fuels, and road use: Opportunities for improving transport tax practice”, OECD Taxation Working Papers, No. 44, OECD Publishing, Paris, https://dx.doi.org/10.1787/e7f1d771-en.
[5] Wappelhorst, S., P. Mock and Z. Yang (2018), Using Vehicle Taxation Policy to Lower Transport Emissions: An Overview for Passenger Cars in Europe, International Council on Clean Transportation, https://theicct.org/publications/using-vehicle-taxation-policy-lower-transport-emissions (accessed on 26 July 2021).
[37] WLTPfacts.eu (n.d.), “What is WLTP: The Worldwide Harmonised Light Vehicle Test Procedure?”, web page, https://www.wltpfacts.eu/what-is-wltp-how-will-it-work (accessed on 26 March 2021).
Notes
← 1. Today, a standard medium-size electric vehicle is still 40% more expensive than a comparable (i.e. similar size) internal combustion vehicle (IEA, 2020[6]).
← 2. As the price of electric vehicles continues to drop (due to a significant decrease in battery cost (IEA, 2020[6])) and taxes and regulations become more stringent, the need for this type of incentive will probably be reduced, or even eliminated.
← 3. In Austria, incentives for the uptake of EVs consist of additional tax reductions and exemptions. France and the United Kingdom have opted for bonus payments and premiums for the purchase of EVs. In France, for example, electric and plug-in hybrid electric vehicles (emitting 20 g/km CO2 or less) benefit from a EUR 6 000 subsidy under the fee-bate scheme.
← 4. Although this might increase road safety problems.
← 5. Compared to the increase in a scenario reflecting current and announced policies (Stated Policy Scenario)
← 6. Higher price trajectories could contribute to decreasing uncertainty to reach net-zero targets, especially under conditions that make challenges to decarbonise higher, such as low oil prices (Kaufman et al., 2020[22]).
← 7. Technological optimism is the idea that the potential to reduce emissions is with technology, most often applied at the level of the element’s level, e.g. vehicles. Fossil fuel solutionism is the idea that, as our fuels are becoming more efficient, they are the bridge towards a low-carbon future (Lamb et al., 2020[40]).
← 8. Transport & Environment recommends the difference between emissions reported and those from on‑board testing to be below 10%.