While understanding of the risk associated with the crossing of climate system tipping points and the potential for cascading effects has considerably increased over the past decade (as reviewed in Chapter 2), mitigation efforts still fall far short of what is needed to avoid the crossing of these thresholds, nor are current adaptation efforts sufficient to manage them. Based on the evidence on tipping points reviewed in the previous chapter, the goal of this section is to provide policy recommendations and insights for climate strategies which appropriately reflect the risk of tipping points. It focuses on near-term policy measures and approaches, including net-zero transition strategies, strategies for building resilience to potential impacts of tipping points as well as on technological approaches to monitoring tipping element behaviour and how they can inform and contribute to mitigation and adaptation responses.
Climate Tipping Points
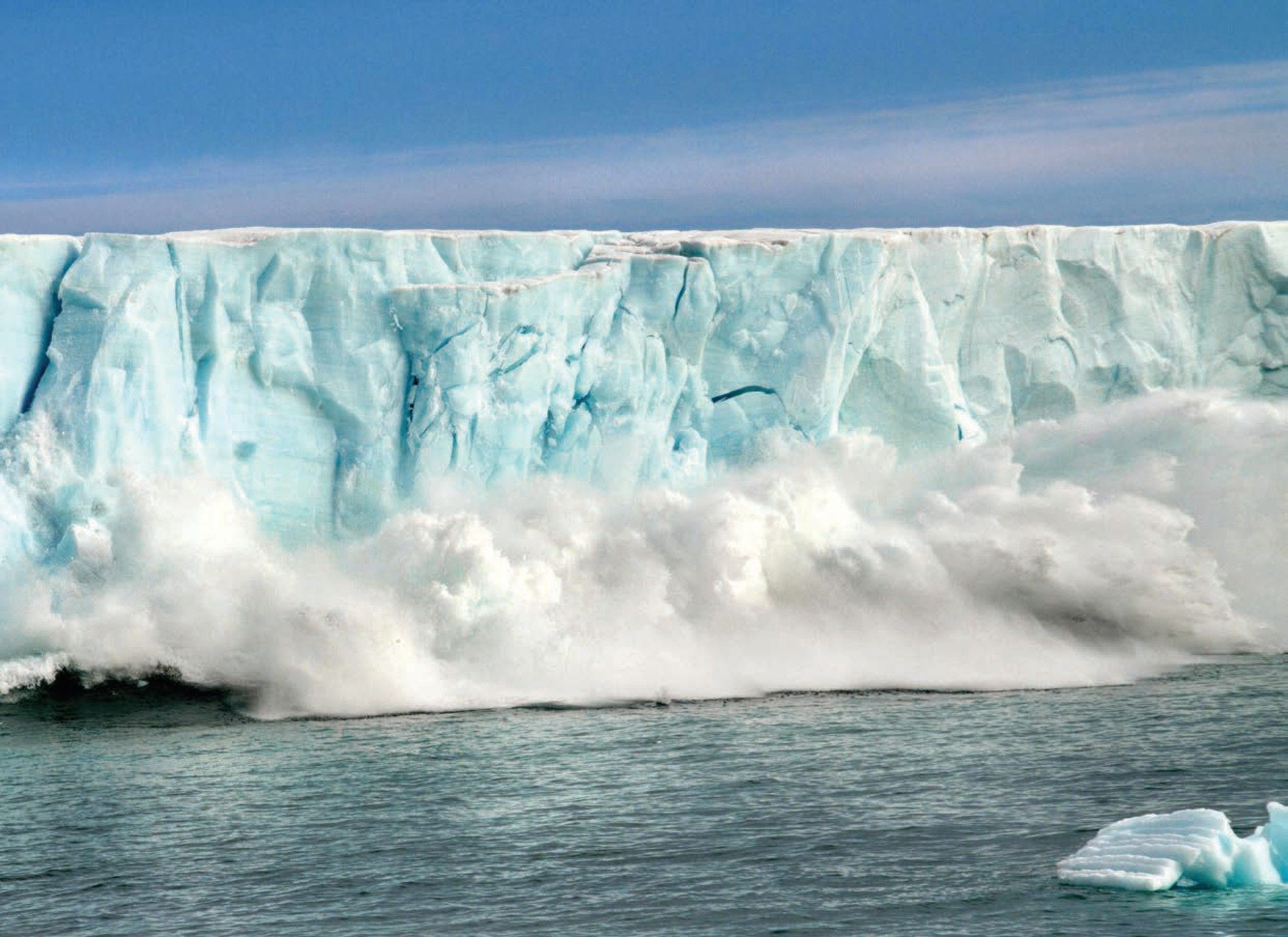
3. Approaches to deal with the threat of crossing tipping points
Abstract
In Brief
Fully integrating the risks associated with climate tipping points into climate risk management strategies requires a precautionary approach to mitigation. Considering that some tipping points may be crossed already between 1.5 and 2°C, such an approach means limiting the temperature increase to 1.5°C, with no or very limited overshoot. Indeed, an overshoot of the 1.5°C limit could result in considerably higher risk of crossing climate tipping points, even if temperatures returned to 1.5 °C levels by the end of the century.
The existence of climate system tipping points therefore effectively limits the number and the shape of emissions pathways towards 1.5°C and renders lenient interpretations of the Paris Agreement goal significantly more dangerous. Limiting warming to 1.5°C with no or very limited overshoot requires a rapid acceleration of near-term action and the transformations needed to reach net-zero CO2 emissions. It is therefore no longer “only” about achieving net-zero emissions by mid-century; rather it is about achieving net-zero with urgent, early and deep reductions in emissions already this decade. Near-term policies in line with meeting current Nationally Determined Contributions (NDCs), put limiting warming to 1.5°C without overshoot out of reach altogether from a biophysical, geophysical, technological and economic feasibility perspective; it is therefore critical that NDCs are strengthened in the very near term, preferably before 2025, and that commensurate policies are implemented at relevant timescales to meet revised targets.
For some tipping elements, other climate and human disturbances, beyond GHG emissions, have the potential to interact with global warming and contribute to surpassing critical thresholds. For example, land use and hydrological changes alongside climate change could lead to widespread dieback of the Amazon forest in the near-term. In the Arctic, wildfires are increasingly contributing to abrupt permafrost thaw and carbon release from boreal forests. While also direct contributors to global warming, the mitigation of deforestation in the Amazon region as well as the management of wildfires in the Arctic region are key measures to reduce the risk of crossing these tipping points.
Given the lower levels of warming at which climate system tipping points may be crossed, some may not be avoided. Adaptation has a crucial role to play in reducing all three main drivers of climate risk, namely managing vulnerability and exposure of populations as well as managing physical hazards directly. Transformational adaptation consists in adaptation that leads to a change in the fundamental characteristics of human and natural systems. It may come with challenges in the short-term as it may disrupt current economic and social systems; in the mid- and long-term however it could generate a range of benefits to human well-being and to planetary health. For example, adaptation measures such as the ban of coastal development, relocation of houses or crop fields to safe areas will very likely need to be considered in order to harness transformation. In addition, transformational adaptation measures that are beneficial or low-cost even in the case where tipping points eventually do not occur can be identified and chosen as “no-regret” policies such as the investment in re-habilitating ecosystems and ecosystems services.
Technological development and innovation have an important role to play in reducing and managing climate risks associated with tipping points. The current understanding of the climate system in general has largely benefited from technological development over the past century, such as in space observation equipment, high computing power, mapping software and telecommunication systems. In particular, technologies for better monitoring and modelling of the climate system will remain essential for detecting early warning signals of the crossing of climate system tipping points. It is difficult to predict the thresholds at which tipping points are crossed, as the driving parameters which induce a shift often show only incremental changes before the system makes a sudden or persistent transition. Strong efforts are being made in climate modelling and remote sensing to improve the detection of early warning signals. Currently these new findings are mostly not yet feeding into risk management strategies and are not informing policy-makers. Greater efforts to systematically make this information available for decision-makers in a digestible manner, will be important to address the threat of tipping points.
The risk of climate tipping points additionally implies a key role for Carbon dioxide removal (CDR) technologies, as scenarios that limit warming to 1.5°C,with no or very limited overshoot all include at least some deployment of CDR, even when stringent demand-side measures are adopted. These technologies are needed mainly in order to help accelerating early deep emissions reductions and to balance out harder-to-abate sectors that continue to act as sources of residual emissions during the first half of the century. There exist today legitimate concerns regarding CDR technologies, in particular relating to the risks associated with bioenergy with carbon capture and storage (BECCS), stemming primarily from its immense demand for land and resulting implications for land-use practices. The potential trade-offs between the risks of employing CDR technologies – and in particular BECCS - and the risks of crossing climate system tipping points, were CDR technologies not to be employed, are today poorly understood. Investments are needed to enhance understanding of these trade-offs and to better evaluate the risks associated with, on the one hand overcoming barriers to scale-up these technologies and on the other, failing to employ them.
3.1. Climate tipping points and climate risk management strategies
Risk management strategies involve “plans, actions, strategies or policies to reduce the likelihood and/or magnitude of adverse potential consequences, based on assessed or perceived risks” (IPCC, 2021[1]). Risks are assessed and evaluated based on their likelihood and potential for impact (Figure 3.1) and to inform risk management, low-likelihood but high-impact outcomes are considered to constitute material risks (IPCC, 2021[1]). Current policy practice has largely focused on higher-probability outcomes (usually lower-impact) and has therefore not effectively applied risk management approaches, despite increasing recognition that climate risk warrants careful risk assessment (King et al., 2015[2]; Sutton, 2018[3]). Given that climate tipping points are generally considered low-likelihood outcomes, they have virtually not been explicitly included in climate policy strategies.
When decisions are made under uncertainty, risk governance processes that facilitate continuous monitoring, evaluation and learning are useful (Klinke and Renn, 2012[4]). Such iterative processes to managing risk can be guided by three closely linked components, namely the characterisation of risks, their evaluation and finally, development, implementation and evaluation of approaches to reduce and manage risks (OECD, 2021[5]). The characterisation of risks is key to risk management strategies, providing direct information to decision makers on the different types of risks and potential impacts they may be facing and informing therefore policy responses to reduce and manage those risks. Having characterised the risk in question, risk evaluation assesses whether this risk is acceptable to the decision maker and their stakeholders1, which is in turn determined by their understanding of the risks, influenced by their values and perspectives. For example, decision makers may consider the acceptability of given risks dependent on a combination of their likelihood and the severity of potential impacts (IRGC, 2017[6]). Figure 3.1 visualises such a framework for risk evaluation, categorising different levels of acceptability whereby; risks are deemed acceptable (where no formal intervention is necessary due to negligible impact and low-likelihood), tolerable (where risks are likely and reduction and risk management measures are available, but where these can be traded-off with non-action considering the costs of the impacts themselves) or intolerable (where potential impacts are too high regardless of their likelihood and risk reduction measures need to be implemented to avoid those risks).
Figure 3.1. Risk evaluation based on probability and potential impact of an outcome
As shown in Figure 3.1, impacts that are potentially “catastrophic”, even if “improbable”, are assessed as intolerable, meaning that decision-makers are not willing to risk them happening and should take measures to avoid them, regardless of cost2. The evidence reviewed in the previous section makes clear that crossing tipping points would entail catastrophic impacts at both regional and global scales, with further implications cascading throughout natural and human systems. In addition, the most recent scientific evidence suggests that important tipping points, including collapse of the Greenland and West Antarctic ice sheets, die-off of low-latitude coral reefs, and widespread abrupt permafrost thaw, may be close to tipping already at current levels of warming [ (McKay et al., 2022[7]), Table 2.1 and Figure 3.2], suggesting even that they may not be “improbable” at all. Tipping points should therefore fall firmly within the “intolerable” risk category and should be managed accordingly.
Global policy efforts, however, are not commensurate with this risks. For example, as discussed in Section 2.4, climate projections informing economic models of potential climate damages only recently started to consider the possibility of tipping points, and thus the economic costs of climate change have often been largely underestimated. This has implications for policy, as policy makers informed by these assessments will choose response pathways that are inadequate for dealing with tipping point risks.
Uncertainties, likelihoods and confidence in results remain important factors determining how risk is perceived. In comparison to previous reports, IPCC AR6 pays special attention to a number of low-probability outcomes that may be associated with high levels of risk, such as low-likelihood high warming scenarios and low-likelihood high-impact events such as tipping points (Chen, 2021[8]). However, the IPCC uncertainty guidance, focusing on likelihoods (based on probabilities), and levels of confidence (based on an evaluation of evidence and agreement across models), are easily misinterpreted. For example, low confidence, in IPCC terms, does not provide confidence on the absence of the potential outcome; rather it provides an indication of the poor state of knowledge on this topic. In addition, an unlikely probability can be associated with high-impact outcomes and be therefore of high importance to risk assessments. Thus, even if climate system tipping points had low probability of being crossed lower levels of warming, which the more recent scientific literature suggests may not be the case, their uncontested potential for catastrophic impacts nonetheless necessitate their incorporation into risk assessments, even if confidence in these outcomes is low.
Historically, however, uncertainties have undermined government responses on climate change. With scientists wary of overstating confidence in their results, confidence levels and uncertainties have often been interpreted as implying climate change may not be an issue after all. In some cases this has been exacerbated by concerted lobbying efforts by vested interests to undermine trust in scientific results. Although Molina and Abadal (2021[9]) argue that stronger likelihoods have since “encouraged stronger action and greater commitment on the part of governments to the mechanisms of international cooperation designed to tackle climate change”, climate tipping points remain low-likelihood events and thus less conducive of action. However, likelihoods in the projection of climate hazards are not the same as climate risk, which is also determined by the vulnerability and exposure of human and natural systems. For effective risk management strategies, policy makers thus need to consider these likelihoods and confidence levels carefully and critically.
Public acceptance is influenced by how uncertainty, likelihood and confidence in results are communicated. Public perception often wrongly attributes higher levels of uncertainty with lower trust in the likelihood of a given outcome, leading to an underestimation of potential risk (Howe et al., 2019[10]). From a risk management perspective, when dealing with uncertainties, one needs to balance the possibilities and consequences of overestimating or underestimating the risk. It is therefore necessary to minimise both false alarms and missed warnings (Shepherd, 2019[11]). Finding a balance between these two extremes is not trivial. There is no single probabilistic threshold that can guarantee a risk is not being over- or underestimated, with multiple factors, including value judgements and risk tolerance, influencing this threshold.
Interestingly, the likelihoods currently informing climate policy responses would be unacceptable in many other areas of life and of decision making. For example, climate pathways considered as consistent with the Paris Agreement are those that provide a “likely” chance, that is a ~67% chance, of meeting the Agreement’s temperature target, leaving a ~33% chance of not doing so. If an aircraft had only a ~67% chance of reaching its destination (and consequently 33% chance of not doing so), this would hardly be considered as an acceptable level of risk. Thus, as with the possibility of an aircraft crashing, low-likelihood, high impact climate outcomes deserve greater scrutiny by policy makers.
In summary, decision making and policy responses to climate change must therefore carefully consider how they address inevitable uncertainties arising from the dynamics of the physical climate system closely interacting with complex and equally dynamic social and economic systems. Uncertainties, likelihoods and confidence statements have been either ignored, not well-understood or misinterpreted in practice, leading often to an underestimating of the gravity of possible climate outcomes Importantly, the new scientific evidence showing that key climate system tipping points are already possible at current levels of warming and likely within the Paris Agreement range (McKay et al., 2022[7]) largely decreases uncertainties in relation to the timescale at which these abrupt changes could start happening. If interpreted correctly, and considering the short timeframe available for action, the uncertainties and ambiguities surrounding climate change projections should amplify – not weaken – the case for strong climate action.
3.2. The role of mitigation in avoiding the crossing of tipping points
Despite progress in collective mitigation plans over the past two decades (see section 3.2.1), even if all announced emissions reduction pledges for 2030 and 2050 are fully implemented, this highly optimistic scenario would still lead to 1.8 °C of temperature increase by the end of the century (Climate Action Tracker, 2021[12]; IEA, 2021[13]). In addition, emission trajectories in line with currently implemented policies are not on track to meet current NDCs and would lead to an increase of about 2.7°C by the end of the century. Despite being within the 1.5-2 C temperature target range of the Paris Agreement, the current level of warming that climate targets for 2030 and 2050 commit the world to, let alone those that currently implemented policies imply, would significantly increase the risk of crossing important tipping points in the climate system. Indeed, Figure 3.2 shows that some tipping elements may already be threatened at current levels of warming and that the probability of tipping elements being triggered increases significantly between 1.5 and 2°C (McKay et al., 2022[7]). This is supported by the IPCC, who project a transition from moderate to high risk for most tipping points at between 1.5-2.5°C of warming (Pörtner et al., 2022[14]). Limiting warming to 1.5°C is thus crucial for avoiding the most dangerous impacts of climate change (IPCC, 2018[15]; IPCC, 2021[16]).
Figure 3.2. Global warming threshold estimates for global core and regional impact climate tipping elements
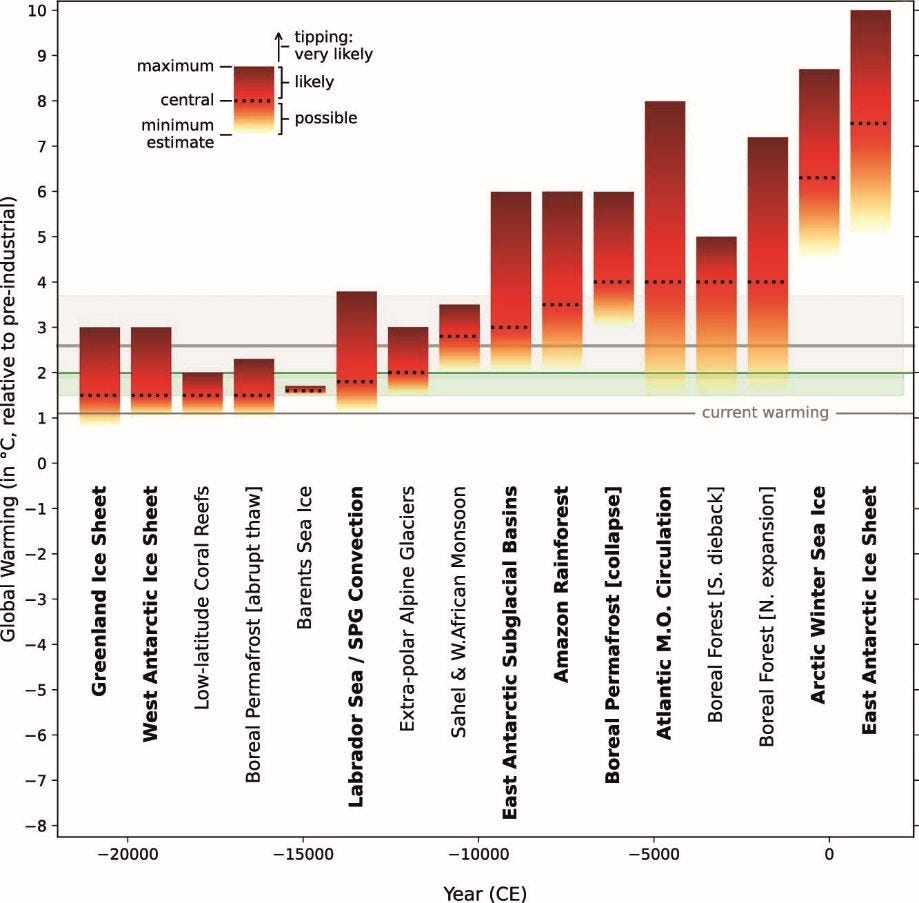
Note: Shadowed in green is the 1.5°C-2°C Paris Agreement range of warming. The shadowed area in grey shows the estimated 21st century warming under current policies (horizontal line shows central estimates). Bars show the minimum (base, yellow), central (line, red), and maximum (top, dark red) threshold estimates for each tipping element (bold font, global; regular font, regional),
Source: (McKay et al., 2022[7])
Under current policy trajectories, projected warming could already exceed 1.5°C, or even 2°C, by mid-century. Indeed the IPCC states that it is “almost inevitable” that the world will exceed the temperature goal of 1.5°C (IPCC, 2022[17]), implying at least some level of overshoot is to be expected. Recent research estimates that if all conditional and unconditional Paris pledges are implemented in full and on time, peak warming could reach 1.9-2°C during the century (Meinshausen et al., 2022[18]). These temperatures could potentially be brought back down to 1.5°C with additional action towards the end of the century, e.g. through net-negative emissions. The overshoot, however, depending on its extent and duration, could still result in crossing irreversible climate tipping points, such as in the melting of the West Antarctica and Greenland ice-sheets and the loss of coral reefs, with severe and irreversible impacts in many natural and human systems [Table 2.1, Figure 3.2 and (McKay et al., 2022[7])].
Fully integrating the risks of climate tipping points into mitigation risk management strategies unequivocally means that a precautionary approach to mitigation should be taken, an approach where global average temperature increase is limited to 1.5°C, with no or limited overshoot. The existence of climate system tipping points limits therefore the number and the shape of emissions pathways towards 1.5°C and renders lenient interpretations of the Paris Agreement goal, as for example allowing for high-overshoot or for warming closer to 2°C at the end of the century, significantly more dangerous and simply incompatible with the Agreement’s resilience goal. This is because in the face of tipping points, simply reaching the temperature target by the end of the century does not ensure a resilient planet and society. The stark reality is that, in the presence of climate system tipping points, a just-below-2°C world could be dramatically different to one where today’s ambition gap is bridged and temperatures are kept at, or below, 1.5°C.
It is also important to note that, if crossed, some climate tipping points would effectively reduce the remaining carbon budget for reaching temperature objectives. Indeed, loss of sea ice and ice sheets leads to a decrease of solar radiation reflection, effectively acting as an amplifier of surface temperatures (see Section 2.3.5). If these tipping points are crossed, emissions reductions would need to be even larger than previously thought to meet stated temperature targets. In addition, permafrost carbon emissions (see Section 2.3.3) have already led to lowering the estimated remaining carbon budgets for achieving the 1.5°C and 2°C objectives (Canadell et al., 2021[19]), even without having reached the threshold for an abrupt tipping point. Since most of these tipping elements are likely to be tipped already within the 1.5°C-2°C range, temperature feedback loops further stress how crucial it is to avoid or limit an overshooting 1.5°C.
Indeed, the science is clear about the fact that collective ambitious climate action keeping warming levels below 1.5°C remains undoubtedly the least uncertain, safest and most cost-effective way to reduce the risks of losses and damages, including the potentially catastrophic impacts of crossing tipping points. As reviewed in Section 2.4, the latest economic assessments show that, when faced with the risk of cascading tipping points and their non-linear and irreversible impacts, the additional costs of implementing stringent climate policies earlier are worth paying. Rapid cuts in emissions now are made all the more necessary by the increasing evidence that some tipping elements may be triggered at lower levels of warming, increasing the urgency of meeting the 1.5oC target with limited or no overshoot. This has important implications for near-term policy, necessitating rapid and deep emissions reductions already this decade. Fully integrating tipping points and their risks within mitigation strategies thus requires countries to significantly increase their efforts, both advancing pledges to levels consistent with the Paris Agreement’s temperature target, and ensuring commensurate policies are implemented at relevant timescales to meet these target. Despite uncertainties and challenges, 1.5°C can still be achieved with very limited or no overshoot (see sub-section 3.2.2). However, as emissions continue to rise, this window of opportunity is closing swiftly.
3.2.1. Collective emissions mitigation efforts have improved but progress falls short
The mitigation of GHG emissions is key in climate risk management strategies, as this is the most direct way to reduce global temperature increase and thus the probability of physical hazards actually occurring. The world has seen a continuous rise in mitigation ambition since 2009, with countries collectively putting forward increasing emissions reductions pledges over the years. Figure 3.3 shows global emissions projections resulting from emissions reductions commitments put forward by countries at three different points in time: in 2009 at COP15 in Copenhagen (yellow wedge), in 2015 at COP21 in Paris (violet wedge) and the latest commitments put forward at COP26 in Glasgow (brown wedge). The figure shows that the level of collective ambition towards 2050 has seen an improvement over the years. In 2009, before any long-term commitment by mid-century was put forward, the so-called Copenhagen pledges were projected to lead to emissions as high as 53-54.5GtCO2-eq by 2050 (Rogelj et al., 2010[20]). Intended Nationally Determined Contributions (INDCs) put forward by countries by COP21 led to emissions levels between 42-51GtCO2-eq (Climate Action Traker, 2015[21]), while the latest assessments of NDCs put forward by COP26 in Glasgow show these emissions could be brought down to up to 25GtCO2-eq, if all long-term targets announced by countries are fully implemented (Climate Action Tracker, 2021[12]).
Figure 3.3. Progression of GHG emissions reductions pledges and projections from 2009 to 2021
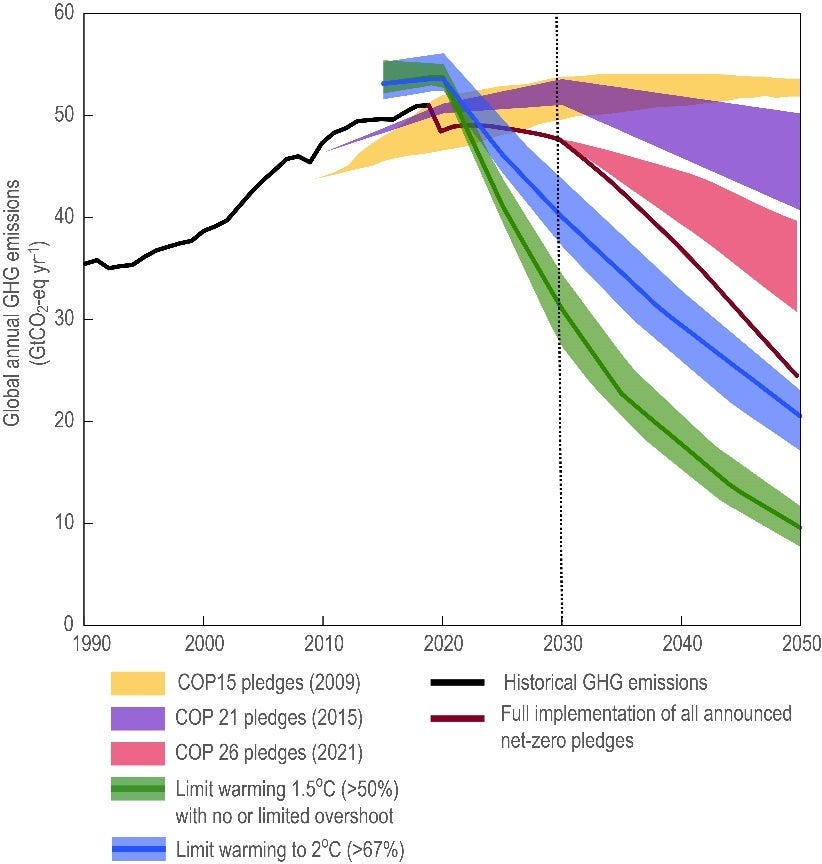
Note: Evolution of GHG emissions resulting from emissions reductions pledges and commitments put forward by countries since 2009. Emissions trajectories in line with pledges made by COP 15 (yellow wedge), COP21 (blue wedge), COP 26 (brown wedge and solid line) are taken from Rogelj et al. (2010[20]), Climate Action Tracker (2015[21]), Climate Action Tracker (2021[12]) respectively. Final historical emissions are taken from (Climate Action Tracker, 2021[12]). The upper end of the brown wedge corresponds to emissions projections based solely on NDCs by 2030 while the lower end also includes long-term binding commitments (Climate Action Tracker, 2021[12]). The brown solid line is considered an optimistic scenario that assumes full implementation of all announced net-zero targets and targets announced in long-emissions development strategies also when those are not yet binding in national law (Climate Action Tracker, 2021[12]). Shaded green and blue areas show GHG emission medians and 25th–75th percentiles for pathways that limit warming to 1.5°C with no or limited overshoot with a 50% chance and pathways that limit warming to 2°C with 67% based on immediate actions from 2020 onwards, respectively (M. Pathak, 2022[22]). Wedges and ranges start at different emissions levels as projections were made at different points starting from historical emissions levels available at the time of the assessment; updates in historical emissions over the years provide adjustments to previous years. This analysis refrains from doing any harmonisation as its goal is to show the progression of emissions reductions commitments over the years and does not make any direct assumption on emissions budgets and implications for resulting temperature increase.
Source: Authors based on data from Rogelj et al. (2010[20]), Climate Action Tracker (2015[21]), Climate Action Tracker (2021[12]) and M. Pathak, (2022[22])
Despite this steady progression of collective mitigation ambition, GHG emissions have continued to rise since 1990 in all major sectors of the economy and in all major groups of GHGs (IPCC, 2022[23]). Figure 3.4 shows that annual average GHG emissions during the past decade (2010-2019) have been higher than in any other previous decade. In addition, emission trajectories in line with currently implemented policies are not on track to meet current NDCs and would lead to an increase of about 2.7°C by the end of the century (Climate Action Tracker, 2021[12]).
Figure 3.4. Total anthropogenic GHG emissions between 1990 and 2019
Although recent emissions increases are in-line with legally binding targets set by countries for 2020, the 2030 targets set in the NDCs fall short of 1.5oC trajectories. Even under an optimistic scenario whereby announced net-zero targets are implemented in full (brown line in Figure 3.3), median warming would reach 1.8°C. Indeed, if 2030 targets pledged in the NDCs are not strengthened, very deep reductions would be required thereafter in order to return to 1.5°C by the end of the century (with a 50% chance), and a high-overshoot would be inevitable (IPCC, 2022[23]).
For indicative purposes only, a trajectory in line with full implementation of net-zero targets currently announced by countries could lead cumulatively to more than 1200GtCO2-eq GHG emissions over the next three decades (M. Pathak, 2022[22]). The least ambitious realisation of COP 26 pledges could increase that cumulative budget to more than 1400GtCO2-eq of GHG emissions. To put that into perspective, the remaining CO2 budget in line with 1.5°C is estimated to be at around 400 [180-620] GtCO2 with a 67% chance. While these budgets are not directly comparable (as the former budgets refer to GHG cumulative emissions and the latter only CO2), it is fair to say that current pledges largely surpass what would be a safe trajectory to 1.5°C. All the more alarming is that emissions from the last decade are roughly the same size as the remaining 1.5°C budget, and emissions today are still increasing, showing that a drastic change in emissions trends this decade is needed (M. Pathak, 2022[22]).
Given this evidence it is clear that mitigation ambition needs to be rapidly increased. With the Paris Agreement only mandating a revision of NDCs every 5 years (starting in 2020), further ambition increases are not expected until 2025. However, given the urgency of the climate problem, particularly in light of the evidence on climate tipping points, steepening emissions reductions trajectories to 2030 is fundamental to remaining within a safe operating space climatically. Governments should therefore step-up in their efforts to revise current climate policy targets immediately.
3.2.2. Key features of mitigation strategies in line with limiting warming to 1.5°C with no or very limited overshoot
In order to avoid the potential catastrophic impacts of crossing climate tipping points, global average temperature increase needs to be limited to 1.5oC with limited or no overshoot. This section reviews key features of mitigation scenarios meeting this target, drawing on work by Riahi et al (2021[24]).
First, limiting warming to 1.5°C, with limited or no overshoot, requires a strong acceleration of near-term policy actions in line with transformations needed to reach net-zero CO2 emissions by mid-century. Typically, net CO2 emissions need to drop by 50% by 2030, and reach net zero in the 2050s (Riahi, 2022[25]). Figure 3.5 illustrates how early, strong action in this very decade becomes imperative for avoiding or limiting overshoot which could trigger tipping points, and emissions pathways that do not make deep cuts by 2030 are ruled out. Alongside reductions in CO2 emissions, achieving rapid reductions in non-CO2 GHGs in this decade, in particular in methane, are also key to constrain the overshoot of 1.5°C. Scenarios that reach 1.5°C of warming with no or limited overshoot reduce methane emissions on average by a third before 2030 and halve them by 2050 (Riahi, 2022[25]). Weak near-term policies, such as those implied by NDCs, put limiting warming to 1.5°C out of reach altogether. Pathways consistent with their NDCs imply higher fossil fuel development until 2030. Accelerated emission reductions after the 2030s would not be sufficient to avoid an overshoot of 1.5°C, and additionally would be made more challenging by the build-up of fossil fuel infrastructure resulting in carbon lock-ins. Considering the risk of crossing climate tipping points, it is crucial that emissions levels implied by current NDCs are not locked in.
Figure 3.5. Implications of global GHG emission trajectories by 2050 for overshooting 1.5°C
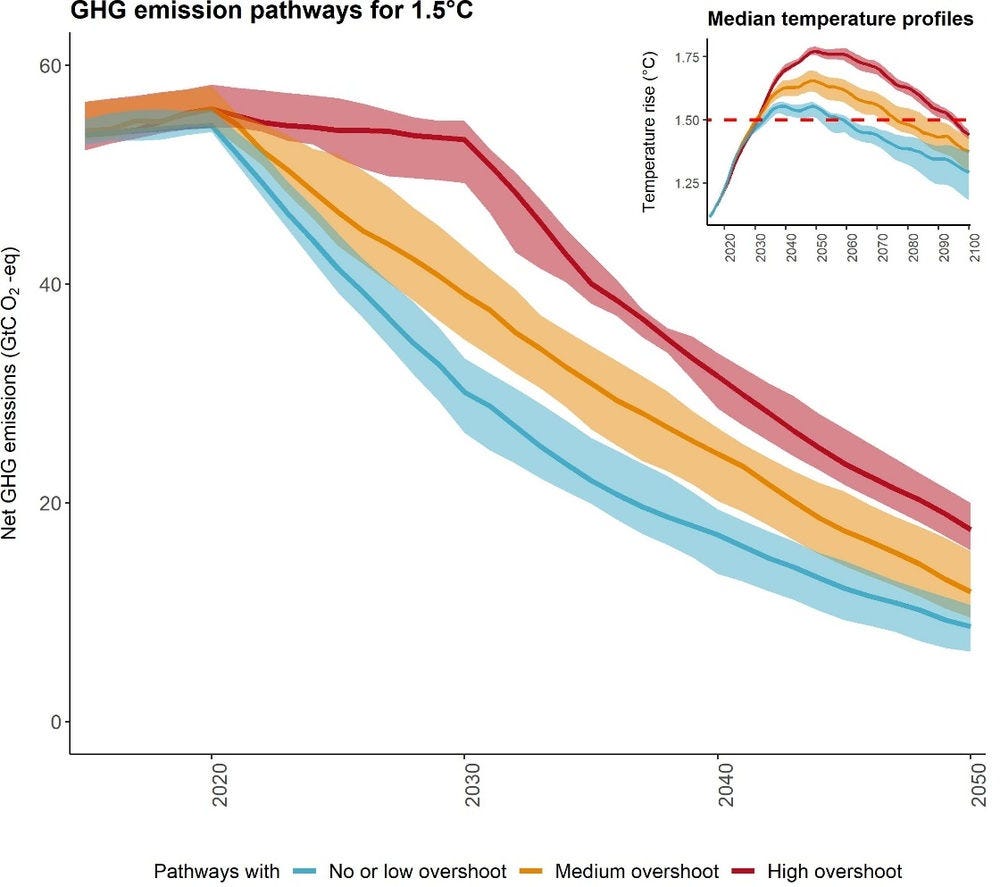
Note: Global net GHG emission pathways (main) and median temperature rise profiles (top right) of scenarios from the IPCC AR6 scenarios database that result in less than 1.5°C of warming in 2100 with a 50% likelihood. Scenarios are sorted into 3 overshoot categories depending on their associated median values of peak warming temperature over this century: no or low overshoot scenarios (median peak warming of 1.6°C or below), medium overshoot scenarios (median peak warming of 1.6°C to 1.75°C) and high overshoot scenarios (median peak warming of 1.75°C to 1.86°C). Lines represent median yearly values and shaded areas represent 25th-75th percentile yearly value ranges for each overshoot category. Net GHG emission values correspond to total Kyoto GHG emissions using the AR6 Global Warming Potential with a time horizon of 100 years (AR6-GWP100) factors to convert emissions into CO2-equivalent values. GHG emissions are calculated by AR6 WGIII using all available gas species reported by the scenario providers as well as infilled emissions species to comprise a more complete basket of Kyoto gases. The temperature rise profiles are assessed by AR6 WGIII using the climate emulator MAGICC. Temperatures refer to Global Surface Air Temperatures (GSAT) and warming refers to the temperature rise above 1850-1900 temperatures. Only scenarios that passed the AR6 vetting process and received a climate assessment are considered.
Source: Analysis made by the authors based on data from the IPCC AR6 scenarios database (Byers, 2022[26]).
Second, all scenarios compatible with 1.5oC with no or limited overshoot rely on achieving net negative emissions through the deployment of carbon dioxide removal technologies, in addition to rapid early emissions reductions already over the next decade. In the scenarios, afforestation, reforestation and BECCS are responsible for the bulk of these negative emissions. Typically, as part of current net-zero strategies, demand-side sectors such as transport, industry and buildings remain emissions sources, while the AFOLU and energy sectors act as sinks. This highlights the importance of considering demand-side mitigation measures in order to limit the level of negative emissions needed. For example, improved energy efficiency, behavioural change and wide-scale electrification will be key to meeting emissions reduction targets.
Third, in order to avoid overshoot, near-term mitigation costs will increase considerably requiring higher upfront investments, but also imposing some short-term costs. Modelling suggests that once net-zero emissions are achieved in the latter part of the century, however, these costs will ease, for example with carbon prices able to decrease to maintain emissions at net-zero balances. This is in turn accompanied by an increase in GDP, with modelling results indicating this rebound in GDP would be much larger than the near-term costs imposed by stringent mitigation policies. In fact, the accelerated near-term transformation in line with avoiding temperature overshoot would have benefits for long-term GDP compared with current policy trajectories, even without accounting for the benefits of avoided impacts, which in the case of tipping points, are very high (see Section 2.4). Importantly, the study finds that the relatively low costs of mitigation implied by NDCs are over-shadowed by considerably higher costs from 2040 onwards until the end of the century.
Fourth, there are large differences across regions regarding the timescales for reaching net-zero, or even net-negative, emissions, with some regions acting as sinks as others continue to emit. Importantly, although such regional differences would pertain the most efficient and effective means of reaching the required targets, these scenarios may not be politically feasible. In fact, achieving such an effective collective solution is challenging as it requires international collaboration as well as markets for cross-regional policy frameworks, which are currently not in place. It is encouraging, however, that net-zero targets in major countries/regions, such as China, the European Union and South Korea, are in line with the pace of transformation required, as depicted in Riahi et al (2021[24]).
The recent Working Group III contribution to the AR6 further highlights the key role systems transformation has to play in limiting warming to 1.5°C with no or limited overshoot. Major transitions in the energy sector are needed, including through the deployment of low-emissions energy sources, switching to alternative energy carriers, energy efficiency and conservation and halting the continued installation of unabated fossil fuel infrastructure. In general, effective mitigation strategies involve all sectors of the economy, and the role of demand-side management measures, including e.g. energy and material efficiency and consumption, electrification and circular material use, cannot be overemphasised. Indeed, all mitigation strategies face implementation challenges, for example technological risks, scaling, and costs. Many of these challenges are however considerably reduced in modelled pathways that assume resources are used more efficiently achieving low demand for resources or those that shift global development towards sustainability, including by reducing inequality (IPCC, 2022[23]).
3.2.3. Mitigation of other drivers and human disturbances contributing to climate system tipping points
As reviewed in Chapter 2, for some tipping elements, other climate and human disturbances have the potential to interact with global warming to contribute to surpassing critical thresholds. For example, land use and hydrological changes coupled with the impacts of climate change could lead to widespread dieback of the Amazon forest in the near-term. In the Arctic, wildfires are increasingly contributing to abrupt permafrost thaw and carbon release from boreal forests. Given there remains the possibility that some tipping points are crossed even at 1.5°C of warming, such additional drivers could prove critical in determining levels of climate risk. Thus, in addition to stringent emissions reductions, a better understanding of these other drivers and increased efforts to mitigate them is required in order to avoid crossing catastrophic tipping points.
As wildfires are expected to increase in severity and frequency in the Arctic, efforts to prevent, monitor and mitigate these will prove crucial in avoiding the risk of crossing tipping points in the Arctic permafrost and boreal regions. Tundra and boreal wildfires are now increasingly driving abrupt permafrost carbon release (Miner et al., 2022[27]), as well as boreal forest losses. Wildfires occurring above the Arctic polar circle in regions where they may not directly threaten human activities or settlements – such as the extensive wildfires observed this summer in Alaska which made 2022 one of the worst years on record (Copernicus EU, 2022[28]) – are usually allowed to burn without implementing monitoring or suppression attempts (Irannezhad et al., 2020[29]). Understanding of the role of the increasingly widespread types of fires in the region is only at its beginning, but their impact on the permafrost carbon feedback and on boreal forest carbon release is considerably larger than on local human activities. As such, Arctic wildfires will require international collaboration in order to better understand their role in crossing Arctic tipping elements, and international efforts to monitor and prevent them.
In the Amazon, deforestation is generating widespread degradation of the hydrological cycle, and could cause the Amazon to pass its tipping point much sooner than if only rising temperatures are taken into account. Additionally, the widespread use of fire to clear vegetation leads to greater vulnerability to fire in subsequent years. A better understanding of the negative synergies between deforestation, climate change and use of fire have led scientists to estimate that the Amazon forest is at risk of shifting to a savannah state at 20-25% of deforestation (Lovejoy and Nobre, 2018[30]). Curbing deforestation so as to keep the deforested area within a safety range is required to avoid crossing such a threshold, as is ambitious and accelerated reforestation in deforested regions of the basin (Lovejoy and Nobre, 2019[31]). Mitigation of deforestation and fire disturbances that could lead the Amazon to a tipping point would also rely on investment in fire control by property holders and governments as well as expanding protected areas (Nepstad et al., 2008[32]).
3.3. The role of adaptation in the context of threat of climate system tipping points
The IPCC defines adaptation in human systems as “the process of adjustment to actual or expected climate and its effects in order to moderate harm or take advantage of beneficial opportunities”. In natural systems, adaptation is “the process of adjustment to actual climate and its effects; human intervention may facilitate this” (IPCC, 2022[33]). Even if global warming levels are kept to 1.5°C with no or limited overshoot, some tipping points which are already possible at current levels of warming (McKay et al., 2022[7]) may be crossed and associated impacts unavoidable, so that even if stringent mitigation efforts to limit climate risk are pursued, adaptation will still be needed (Ara Begum, 2022[34]).
It is estimated that currently 3.3 to 3.6 billion people are highly vulnerable to climate change (IPCC, 2022[33]). This number would increase significantly if tipping points were crossed, with most regions and people affected in some way by the associated physical hazards including extreme temperatures, higher frequency of droughts, forest fires, unprecedented weather, food insecurity and faster sea-level rise to name a few (see Chapter 2). Adaptation has a crucial role to play in reducing all three main drivers of climate risk, namely managing vulnerability and exposure of populations as well as managing physical hazards directly, having a crucial role to play in responding but also preparing systems to the potential impacts of tipping points.
The systemic scope and severity of impacts associated with climate system tipping points may impose both soft and hard limits on possible adaptation responses, whereby “soft limits are those for which no further adaptation options are currently feasible but might become available in the future, and hard limits are those for which existing adaptation options will cease to be effective and additional options are no longer possible” (Pörtner et al., 2022[14]). For example, increased sea-level rise due to the tipping of cryosphere points rendering several small islands uninhabitable poses a hard limit to adaptation, and a soft limit to adaptation in other coastal areas where coastal livelihoods would be rendered unsustainable (Mechler et al., 2020[35]). What is more, the potentially abrupt nature of tipping point impacts could render the time available for human systems to adapt too short to allow communities or societies to identify, develop and adopt ad-hoc solutions to adapt to these impacts. Taking into account climate tipping points and the interactions and reinforcing dynamics among ecological, social, and climate processes is therefore crucial for effective and long-term adaptation planning and implementation (Cai et al., 2015[36]; Lontzek et al., 2015[37]; Steffen et al., 2018[38]; Dietz et al., 2021[39]).
This section considers how adaptation efforts can account for the risk of crossing climate tipping points, focusing on the following three aspects. First, the section considers the role of transformational adaptation in building systemic resilience to tipping point impacts. Second, the section considers the importance of monitoring and measuring progress in adaptation for informing adaptation efforts. Third, the section considers the need for new approaches for dealing with uncertainty in decision making.
3.3.1. The role of transformational adaptation in reducing the risks of climate tipping points
Transformational adaptation is that which leads to a change in the fundamental characteristics of human and natural systems so that the capacity of these systems to cope with potential hazards is increased (IPCC, 2022[33]). Considering the scale and magnitude of impacts associated with the crossing of tipping points, fundamental changes in systems will be unavoidable to adapt to those impacts. More effective however is to prepare to those impacts through changing these key fundamental attributes of socio-economic systems, through energy, land-use, infrastructure, industrial and societal systems transitions before, the occurrence of these impacts through transformational adaptation.
This report therefore argues that the scale and magnitude of tipping point impacts necessitate a transformational, beyond incremental, approach to adaptation. Incremental adaptation refers to measures that target specific systems components, safeguarding these from given climate risks, and ensuring system functioning into the future (Loginova and Batterbury, 2019[40]) (Kates, Travis and Wilbanks, 2012[41]). In comparison, transformational adaptation can be more effective in building resilience because it implies changes in the fundamental characteristics of the human and natural systems so that the capacity of these systems to cope with potential hazards is increased (IPCC, 2022[33]). The IPCC defines resilience as “the capacity of social, economic and ecosystems to cope with a hazardous event or trend or disturbance, responding or reorganising in ways that maintain their essential function, identity and structure as well as biodiversity in case of ecosystems while also maintaining the capacity for adaptation, learning and transformation” (IPCC, 2022[33]).
The latest Working Group II contribution to the IPCC AR6 considers transformational adaptation as “one component of climate resilient development in which adaptation, mitigation and development solutions are pursued together to exploit synergies and reduce trade-offs among these actions” (Ara Begum, 2022[34]) (Box 3.1 discusses in more detail some of these synergies and trade-offs). This is also reflected in the IPCC WGIII report on mitigation, in that, alongside its role for mitigation, the transformation of whole systems also has a key role to play in achieving transformational adaptation (Lecocq, 2022[42]). While transformational adaptation comes with challenges in the short-term by potentially disrupting current economic and social systems, in the long-term it could generate a range of benefits to human well-being and to planetary health (Schipper et al., 2022[43]) which could end up saving communities and the environment.
In light of the threat of climate system tipping points, transformational adaptation could necessitate stringent and even drastic measures to reduce impacts and avoid losses. Many responses in line with transformational adaptation are technological – for instance, implementing water capture and storage solutions in areas at risk of drying. They can also be behavioural, or alternatively include fundamental changes in institutional arrangements, priorities, and norms (Kates, Travis and Wilbanks, 2012[41]). In addition, transformational adaptation measures can target the spatial development of human activities, including through managed or strategic retreat of communities and settlements, relocation of assets and infrastructure or long-term spatial planning and urban and agricultural zoning. Other examples include cooperative governance such as international cooperation among governments to manage migrations, the deployment of Nature-based Solutions (NbS), and livelihood transformation in land systems (New, 2022[44]).
Concrete examples for transformational adaptation in response to the risk of crossing climate system tipping points have been explored in the literature. First, the disintegration of ice-sheets would result in considerable sea-level rise, posing an existential risk to low-lying coastal areas and countries, making stringent and transformational adaptation measures unavoidable. For regions with low tolerance to uncertainty, where key economic sectors are at risk, credible adaptation responses would need to consider these plausible high-impact scenarios of SLR and enforce drastic measures such as no-building zones and planned retreat from exposed areas (Haasnoot et al., 2019[45]). In another instance, studies suggest that energy planning and zoning in the Amazon region, which is currently heavily reliant on large-scale hydropower, needs to consider the potential impacts of a dry-state Amazon forest on the hydrological cycle of the region and plan for a decentralisation of energy production, a diversification of energy sources focusing on small-scale hydropower and solar power, and investing in energy saving (Lapola et al., 2018[46]). Taking a transformational approach to adaptation in that region would also mean protecting the agricultural sector through livelihood transformation by encouraging farmers to switch to crop varieties and livestock adapted to drier conditions (ibid).
Transformational adaptation measures that are beneficial or low-cost even in the case where tipping points do not occur can be identified and chosen as “no-regret” policies (Heltberg, Siegel and Jorgensen, 2009[47]). Some may come with large co-benefits by contributing to reductions in GHG, supporting the advancement of other sustainable development goals – e.g. energy and water access – and building systems’ and populations’ resilience to future climate shocks. This is especially the case for, the investment in re-habilitating ecosystems and ecosystems services and the implementation of NbS. For example, coastal ecosystem conservation helps ensure coastal protection against increased risk of SLR from ice sheet tipping points, but may also improve food access from fisheries, flood regulation, biodiversity conservation, as well as provide recreation and cultural benefits (Schipper et al., 2022[43]). Importantly, NbS are also effective in accelerating mitigation efforts. Forest maintenance and restoration is a key carbon sequestration measure, and has a range of potential sustainable development co-benefits, including food provision, fuel provision, job creation, biodiversity conservation, air quality regulation, and water and soil conservation (Schipper et al., 2022[43]). In addition, in the Amazon, investing in forest protection would contribute to mitigate the risk of a dieback tipping point (see Section 3.2.3). A further example of no-regret policy includes energy decentralisation which can help ensure universal access to energy while increasing resilience in the case of adverse impacts from a tipping point in the Amazon region.
Many adaptation initiatives today prioritise near-term climate risk reduction – also largely in line with short political cycles - which may constrain opportunities for transformational adaptation (IPCC, 2022[33]) and clearly indicates that much more needs to be done. Even if the Paris Agreement’s temperature target are met, it is likely that some transformational adaptation will be needed (Ara Begum, 2022[34]). Thus, in addition to pursuing stringent mitigation efforts to limit climate risk, transformational adaptation efforts must also be pursued to ensure resilience to unavoidable impacts, including those associated with the crossing of tipping points. If mitigation efforts fall short of the 1.5°C target, transformational adaptation will be all the more important as impacts become more severe, and the risk of crossing tipping points increases (Ara Begum, 2022[34]). Implementing transformative actions that also support sustainable development goals in response to the risk of climate tipping points being crossed provides a key opportunity for climate resilient development.
Box 3.1. Linkages between mitigation, adaptation and sustainable, climate-resilient development
The recognition and appropriate assessment of climate risks, including the risks of crossing tipping points can strengthen adaptation and mitigation actions that lead to the transformation of key systems, in line with sustainable development and with strengthening the resilience of ecosystems and society. Accelerated, effective and equitable climate action for both mitigation and adaptation is crucial for achieving sustainable climate-resilient development. If not addressed, climate change will negatively impact, directly or through its cascading effects, the achievement of sustainable development.
For example, climate change will have negative impacts on the health and livelihoods of people around the world and on ecosystem health and biodiversity, both of which are the heart of the Sustainable Development Goals (SDGs). Climate action, on the other hand, may lead to improved energy efficiency and the use of renewable energy, the reduction of air pollution, shifts to more balanced, healthier diets, reforestation or forest conservation and avoided deforestation, all of which are examples of synergies between climate mitigation and sustainable development. There are, however, also potential trade-offs here that are relevant when dealing also with the threat of climate system tipping points, particularly considering land-use based mitigation such as re- and a-forestation, in terms of employment and the affordability of food, energy and water. Carbon capture and storage technologies also entail potential negative impacts on clean water and sanitation. While synergies largely outweigh trade-offs, the latter need to be managed through careful planning and implementation of climate policies. Maximising synergies and avoiding or managing trade-offs pose particular challenges for developing countries and vulnerable populations. Indeed, there is a strong link between sustainable development, vulnerability and climate risk.
Limited economic, social, and institutional resources often lead to higher vulnerability and lower adaptive capacity in particular in developing countries. Even if global mitigation targets are achieved, there will be a large need for financial, technical and human resources for adaptation. In addition, some mitigation options can lead to reduced adaptive capacity as for example, in the large-scale or poorly planned deployment of bioenergy and afforestation of naturally unforested land. By contrast, there are a range of response options that maximise both mitigation and adaptation, such as sustainable urban planning and infrastructure design including green roofs and facades, agroforestry, and the restoring natural vegetation and rehabilitating degraded land. Coordinated and coherent policies integrating mitigation and adaptation policies across sectors can maximise synergies and therefore enhance support for climate action, but this requires resources in social and institutional systems.
Enhanced mitigation and action in line with sustainable development will have distributional consequences within and across countries. Countries vary in their level of development, but also in their social, economic, cultural, environmental and political contexts. Sustainable development must take into account these differences and their resulting needs as regards development outcomes. Rapid and broad mitigation actions may result in disruptive changes with distributional consequences including the shift of employment and income from emissions intensive sectors to low-emissions activities. Low-emissions development can however create opportunities to build skills and create jobs. Applying just transition principles and integrating equity, justice and gender-equality considerations into policy packages at all scales can reduce challenges associated with the necessary shift towards low-emissions pathways.
Source: (IPCC, 2022[23])
3.3.2. The importance of measuring progress on adaptation
There is sparse evidence that existing adaptation actions actually reduce climate risk (O’Neill, 2022[48]). As with mitigation efforts, although adaptation actions are increasingly implemented worldwide, the rate and scale of adaptation progress falls short of what is needed to keep up with mounting climate risks (UNEP, 2021[49]). National-level adaptation planning has seen progress over the recent years, with an increase in the development of adaptation planning instruments and positive trends across almost all criteria of adequate and effective adaptation planning (UNEP, 2021[49]). Data on the effectiveness of implemented adaptation activities in actually reducing climate risk, however, is limited. Current adaptation implementation rates are unlikely to keep pace with mounting impacts from climate change (UNEP, 2021[49]). While progress on adaptation planning and implementation has been observed in all sectors and regions, that progress is also unevenly distributed across households, geographies and income levels with most observed adaptation being fragmented, small in scale, incremental and sector-specific (IPCC, 2022[33]).
This highlights the need to strengthen the understanding of how adaptation actions contribute to effective policy outcomes, with the support of robust monitoring and evaluation frameworks at project, local, national and international levels. Indeed, Article 7 of the Paris Agreement requests countries to engage in “monitoring and evaluating and learning from adaptation plans, policies, programmes and actions” (Paris Agreement, 2015[50]). According to the IPCC, in the context of adaptation, M&E consists of “systematic process of collecting, analysing and using information to assess the progress of adaptation and evaluate its effects - e.g., risk reduction outcomes, co-benefits and trade-offs - mostly during and after implementation” (New et al., 2022[51]). M&E is particularly important as climate change and associated impacts are dynamic processes that will change in the future. Hence, identifying successes and failures enables learning, and continuous monitoring or adaptation measurement allows efforts to be adapted to changing circumstances (Box 3.2). Moreover, under deep uncertainty, there is growing evidence that effective implementation strategies require M&E in order to judge outcomes against possible futures scenarios (OECD, forthcoming). This is particularly relevant in the context of the risks of climate system tipping points. In order to adequately consider the risk of climate tipping points, M&E systems need be informed by the best available science on tipping points, including information on potential hazards associated with crossing tipping points. Particularly important here is information on the early detection of tipping points and the thresholds beyond which they are triggered [ (Bloemen et al., 2017[52]), further explored in Section 3.4.1].
Box 3.2. Adaptation Measurement – knowing when to adjust adaptation actions in an uncertain future climate
Adapting to a changing climate inherently deals with significant uncertainty. It is an ongoing process of adjustment to the evolving impacts of climate change. These impacts are characterised by uncertainty in the response of the climate system to greenhouse gas emissions, in the feedback loops produced in environmental, economic and social systems, as well as in the interconnected and cascading effects, such as those produced by tipping points, that may require unprecedented adaptation, or even demonstrate the limits of adaptation. Adaptation measures that may be effective at a given point in time might be insufficient at another, especially considering mounting climate impacts. As future impacts from climate change, including those resulting from the crossing of climate system tipping points, are difficult to predict, regularly measuring whether adaptation policies are sufficient and effective in reducing climate risk is critical to mitigate losses and damages.
Although progress has been slow, countries increasingly adopt adaptation measurement as part of implementing National Adaptation Strategies (NAS) and Plans (NAPs). Today, 19 OECD countries measure progress in the implementation of adaptation policies. Adaptation measurement entails setting objectives that can be linked to adaptation policy efforts, and can be measured in terms of inputs (e.g. human, financial and technical resources) and outputs (e.g. the deliverables resulting from a policy action). More advanced measurement efforts seek to evaluate whether these actions are effective in reducing climate risk and can be attributed as a result of the roll-out.
For example, in the United Kingdom, the Climate Change Committee, an independent body, conducts the Independent Assessment of UK Climate Risk. It analyses key current and future climate risks, documents the efforts of building climate resilience to date and recommends adaptation actions needed in the coming five years. Its analysis is based on current and planned policies as well as on economic sectors in which most impacts are expected (e.g. infrastructure or the natural environment). Every year, based on the risk assessment, the Committee measures progress in the implementation of adaptation actions. The annual progress report identifies the extent to which adaptation planning is taking place, whether implemented plans consider the key climate risks identified as well as trends in factors contributing to climate risks. Its work is based on adaptation indicators, including vulnerability, exposure, adaptation actions and impact to help assess the effectiveness of the UK’s National Adaptation Plan, in response to the widening adaptation gap identified in the climate risk assessment process.
Over time, adaptation measurement can iteratively inform decision-making, as an adaptive management practice that enables countries to be alerted when adaptation action is insufficient, to protect communities and economics from unfolding climate risks, including any unexpected cascading impacts, or impacts associated with certain tipping points. For this to work, the constant monitoring of the level and distribution of climate risks is necessary as well as an understanding of the effectiveness of current adaptation measures.
Note: Based on the IPCC’s framework, climate risk is composed of exposure, vulnerability and hazard.
3.3.3. Dealing with scientific uncertainty when developing adaptation strategies
Global Climate Models can be highly effective tools that provide a range of valuable information for adaptation in light of the threat of tipping points. As reviewed in Chapter 2, such models provide information that can strengthen collective understanding of potential futures and therefore of how decisions today can contribute to reducing the risk of climate change tomorrow (OECD, 2021[5]). However, they entail considerable uncertainties in relation to the projection of climate impacts that risk being misinterpreted by decision makers. As reviewed in section 2, the projection of impacts and cascading effects associated with climate tipping points are also subject to considerable uncertainties which only addto the adaptation challenge.
Approaches to decision-making under uncertainty have been developed and have been characterising adaptation planning and decision making. One example is the use of “narratives” which treat uncertainty in less rigid frameworks than traditional risk assessment methods and provide scientifically sound qualitative descriptions of plausible low-likelihood, high-impact future world evolutions and are particularly important in providing a better understanding of the risks and potential impacts associated with climate system tipping points. Dessai et al. (2018[60]), for example, use an approach, informed by climate processes and expert elicitation, to build six narratives of future regional precipitation change applied to the Indian Summer Monsoon. The authors show that climate process-based expert elicitation of narratives have the potential to inform regional and local risk assessments and adaptation decisions when future climate uncertainty is large3. Shepherd et al. (2018[61]) argue that this narrative or ‘storyline’ approach is an effective way of linking the physical and human aspects of climate change. They suggest four main reasons supporting their use:
improving risk awareness by moving away from a probabilistic framing of climate impacts towards an event-oriented framing, which captures more directly how people perceive and respond to risk;
strengthening decision-making by allowing policymakers to work backward from a particular vulnerability or decision point and combining climate change information with other relevant factors to address risk;
allowing the use of more credible regional models in a conditioned manner by providing a physical basis for partitioning uncertainty, and;
avoiding false precision and surprise by exploring the boundaries of plausibility.
Such storyline approaches have also been suggested as ways to improve the contributions of the IPCC on informing climate risk assessments, particularly for low-likelihood, high-impact events such as tipping points (Sutton (2018[3]). One example explored is the potential for abrupt change in the AMOC during the 21st century (among others). While low-probability, policymakers should nonetheless concretely consider the potential for high-impact hazards associated with an abrupt transition of the AMOC during this century in climate risk assessment which are explored in these storylines (Sutton, 2018[3]). Indeed, the latest WGI contribution to the AR6 explicitly assesses low-likelihood high-impact outcomes by using physical storylines, in particular in the context of “high-warming scenarios” (Arias et al., 2021[62]). These scenarios consider the low-likelihood, high-impact outcome that expected warming levels at given concentrations of GHG are surpassed (OECD, 2021[5]). Such a scenario would lead to far more severe global and regional impacts than projected, including higher risks of crossing tipping points (Arias et al., 2021[62]). Through detailing such a future through a storyline approach, the IPCC report highlights the importance of such scenarios despite associated uncertainties, and thus enables them be taken into account into climate risk assessment informing adaptation responses.
Another effective approach of decision-making under uncertainty, the Dynamics Adaptation Policy Pathways (DAAP), takes into account the multitude of uncertainties decision makers need to consider, e.g. in how climate change will unfold or how societies and economies will evolve (Haasnoot et al., 2013[63]). This approach is based on the establishment of a framework for action that is informed by a strategic vision of the future and guided by short-term, flexible actions that can be adjusted to reflect changing circumstances. DAAP is therefore a proactive decision support method, where decisions are taken to prevent, rather than to respond to, critical changes. As such, DAAP supports the development of adaptation plans where measures can be adjusted before crossing a tipping point (Franzke et al., 2022[64]).
3.4. The role of technology in addressing the risks of climate system tipping points
Technology has a key role to play to reduce and manage climate risk. Risk governance processes need to include mechanisms facilitating continuous monitoring, evaluation and learning, particularly when decisions are made under uncertainty (OECD (2021[5]) (Klinke and Renn, 2012[4]). Since there is no prior experience in dealing with climate system tipping points risks, such processes need to rely on emerging understanding of risks. This can be guided by the following components (OECD, 2021[5]): (1) characterisation of the risks; (2) evaluation of the risks and (3) development, implementation and evaluation of approaches to reduce and manage the risks. As discussed in previous sections, these are key components for incorporating the risk of tipping points within both mitigation and adaptation policies. Technology has a crucial role to play here and this section provide an overview of how different technologies can support each of these components specifically when dealing with the risks of tipping points.
3.4.1. Role of technology in informing the characterisation of risks of crossing climate system tipping points
As reviewed in Chapter 2, understanding and characterising the risk of crossing tipping points, their potential impacts and cascading effects, is a complex scientific problem. The current understanding of the climate system as whole has largely benefited from technological development over the past century. In particular, advances in space observation equipment, high computing power, mapping software and telecommunication systems, provided essential tools to advance observational data collection and climate modelling. These tools are and will remain essential for the identification of climate system tipping elements and their behaviour under different warming scenarios.
In order to quantitatively understand features of the Earth’s system relevant to climate system tipping points, physical theories and Earth observations are turned into models that represent these key features and their interactions. Earth is more extensively and systematically observed today than at any other time, benefitting from advances in satellites and remote sensing (Agapiou, 2016[65]; Reichstein et al., 2019[66]). The resulting data is crucial for the calibration and evaluation of climate models and therefore contributes to characterising risks associated with climate change, including the risks of climate system tipping points.
Weather and climate information services (WCIS) provide information on past, present and future states of the atmosphere and so inform climate policy decision-making (Allis et al., 2019[67]). In the presence of climate system tipping points, such services are crucial for monitoring the potential approach of tipping points and the impacts resulting from their crossing. WCIS are also essential for identifying and assessing options for reducing and managing risks as well as for monitoring the performance of implemented measures. Research suggests that, while weather services are well established, climate services informing longer timescales are less so, despite major progress in recent years (Hewitt et al., 2020[68]). Continued investments in the WCIS value chain (Figure 3.6) are necessary to ensure reliable delivery of weather and climate information. It is crucial that these products remain relevant, accessible and credible to a wide audience of decision makers, clients and communities (WMO and GFCS, 2019[69]).
Earth observations from satellites provide information on climate and climate change, relying on a complementary mix of satellite- and surface-based measurements. Such data are also crucial for supporting early warning systems (EWS) in the context of climate system tipping points, which are further discussed below. For instance, the World Meteorological Organization Global Observing System (WMO GOS) encompasses both surface- and space-based observations essential for understanding the Earth system and for the production of WCIS. Indeed, satellite data from the WMO GOS provide 90% of the data for Global Numerical Weather Prediction, which underpins most Earth system modelling approaches (ITU, 2020[70]).
Figure 3.6. Weather and climate information service value chain
The physical hazards resulting from the crossing of tipping points interact with the vulnerabilities of exposed communities and natural systems to determine risk. In this sense, technologies for the collection of data on exposure and vulnerability are crucial for the characterisation of risks. Understanding societal vulnerability requires granular socio-economic data and assessments of the impact of climate hazards on people’s livelihoods and health, which is often not directly quantifiable. Geospatial data, analysis and visualisation tools, including satellite imagery, aerial or unmanned aerial vehicle (UAVs or drones) images, mobile communications, and social media can provide valuable information on exposure and vulnerability (OECD, 2021[5]). The use of such technology, however, requires real-time capabilities to monitor the exposure of people as well as advanced data processing capacities. Coupling high-resolution geospatial data on the patterns of exposure and vulnerability with artificial intelligence capabilities may thus help guide response design and implementation (OECD, 2021[5]).
At the international level, Shared Socio-economic Pathways (SSPs) have been developed to describe different potential development pathways making assumptions on variables such as level of economic growth, poverty and demographic change which are closely linked to vulnerability and exposures. While they are scenarios and by no means direct predictions of the future, in conjunction with climate scenarios, they provide a good indication of how the three drivers of risks can interact to determine impacts (O’Neill et al., 2017[73]).
Early warning systems for characterising the risks of tipping points
Technologies for better monitoring and modelling of the climate system will be essential for characterising how climate-related hazards resulting from the crossing of climate system tipping points may evolve over time and space. Thus, they can more broadly identify features of systems approaching a tipping point. In this sense, high-quality scientific data in an appropriate frequency and resolution are key to understand, monitor allow for early warning of tipping elements. Due to the need to assess the complex interactions between natural variability and anthropogenic forcing (Swingedouw et al., 2020[74]), detecting early warning signals is challenging but any information provided is invaluable for the understanding of how the Earth system is approaching some of these critical transitions (Ditlevsen and Johnsen, 2010[75]; Lenton, 2011[76]; Swingedouw et al., 2020[74]; Bury, Bauch and Anand, 2020[77]; Rosier et al., 2021[78]). The current state of early warning systems for climate system tipping points and potential avenues for improving them are reviewed below.
It is difficult to predict the state at which tipping points are crossed as the driving parameters which induce a shift often experience only incremental changes before the system in question makes a sudden or persistent transition. One method to help detect early loss of system resilience consists in Early warning indicators (EWI), which are statistical methods that can quantify the loss of temporal or spatial resilience, signalling the approach of crucial thresholds (Gsell et al., 2016[79]; Lenton, 2011[76]; Boulton and Lenton, 2015[80]; Boers, 2021[81]). Recent analysis shows that deep-learning, or artificial intelligence algorithms, can find more reliable early warning signals than more established methods as they use extra information from patterns in the data (Bury et al., 2021[82]).
Another method that has proven to be successful in detecting early warning signals of abrupt shifts in climate state in climate modelling and observational datasets is a machine-vision approach to automatically detect edges (Bathiany, Hidding and Scheffer, 2020[83]). This approach can quantify abruptness, provide details to help understand the causes of the shift, and assess some uncertainties of climate events. This method has proven to be effective in identifying “climate surprises” (Bathiany, Hidding and Scheffer, 2020[83]), showing, for example, abrupt shifts related to the loss of sea ice and permafrost in the Arctic (Bathiany, Hidding and Scheffer, 2020[83]).
Almost all existing methods for monitoring early warning signals rely on data on the present state of the climate through direct and remotely sensed observations and play a crucial role in the characterisation of tipping point risks. While remotely sensed observations have been significantly improved over the years (Guo, Zhang and Zhu, 2015[84]; Reichstein et al., 2019[66]), better monitoring technologies are needed to improve high-level observation of disturbances in spatial patterns related to fragile transitions in systems. Ideally, an international scientific programme could focus on monitoring, modelling and developing potential EWIs for a range of tipping elements. Progress in this direction seems to be underway. In early 2021, for example, the ESA Climate Office convened a forum on Remote Sensing of Tipping Points in the Climate System; the forum was hosted by the International Space Science Institute (ESA, 2021[85]). Participants recognised that while the spatial resolution of satellite datasets and the resolution across the frequency spectrum are both improving, these improvements have associated challenges, such as the need to bridge scale differences between observations and models. Participants also noted the importance of methodologies to take advantage of the high resolution imagery available in remote sensing datasets to study spatial patterning and nonlinear disturbance effects.
Observational data on abrupt climate changes that occurred in the geological past is also needed to improve the ability of models to characterise, and therefore predict, couplings and feedbacks in the Earth system and the potential for tipping points (Lenton et al., 2019[86]). Constraining, for example, current ice sheet models with new historical data will progressively allow reanalysing past ice sheet mass loss. This can contribute to enhancing our understanding of the underlying processes of ice sheet melting and improve the ability for models to reproduce past changes, increasing the reliability of their projections (Lenton et al., 2019[86]; Swingedouw et al., 2020[74]; Brovkin et al., 2021[87]).
In general, there are a number of valuable efforts by the climate modelling community and remote sensing experts in improving the detection of early warning signals. It is less evident how these new findings feed into risk management strategies, informing courses of actions contemplated by policy-makers. Greater efforts to systematically make this information available, in a digestible manner, for decision-makers need to be made if the threat of tipping points are to be addressed.
3.4.2. Role of technology in informing the evaluation of risks of crossing climate system tipping points
Technology has an important role to play in risk evaluation (Figure 3.1). Risk evaluation involves a broad range of values, including societal values, economic interests and political considerations, which influence perceptions of risk. Given differences in values across individuals, communities and cultures, it is crucial that the approach to risk evaluation is participatory through, for example, the creation of community risk assessment tools. They can create an exchange of information that informs decision makers on how society judges risks and brings to light communities’ perspectives on increased climate risks to livelihoods and systems (van Aalst, Cannon and Burton, 2008[88]). The methodology may involve a range of hard and soft4 technological tools, including risk mapping, focus group meetings, surveys and discussions, and interviews.
As an example, digital community risk maps facilitate risk-knowledge co-generation informing the evaluation of climate risk in general and also generating information that is useful for managing the risk of crossing climate system tipping points. For example, the Risk Geo-Wiki portal established by the International Institute for Applied Systems Analysis, provides a two-way information exchange between local knowledge and expert-sourced knowledge of risk (Geo-Wiki, n.d.[89]). It allows for a community-based participatory mapping process where community members can provide, for instance, hand-drawn maps of the locations of critical infrastructure, emergency shelters and other community resources. The information is then digitalised and can be further updated developed by local stakeholders. These digitalised maps can be overlaid with satellite imagery to better visualise and aid in planning, designing and developing initiatives. The portal has been used in Nepal, Peru, Mexico (Mechler et al., 2018[90]).
With advances in geospatial technologies, qualitative local knowledge can now be translated into mathematical models to evaluate quantitatively potential outcomes of climate change (Hemmerling et al., 2019[91]). The process can therefore incorporate localised knowledge into usable quantitative datasets that can serve multiple purposes in the planning process. Such quantification can, for example, be used to identify particularly vulnerable or exposed social or cultural groups, and provide useful information for reducing and managing risks in geographically targeted evidence-based planning strategies. This allows policy makers to make informed decisions, such as on the adoption of new adaptation and resilience plans, even where traditional quantitative data informing risk assessments is lacking (Cornforth, Petty and Walker, 2021[92]).
3.4.3. Role of technology in the development and implementation of approaches to reduce and manage risks of crossing climate system tipping points
Technology has a crucial role to play in climate strategies, helping to avoid and reduce risks through mitigation and adaptation. Without aiming to be comprehensive, this section discusses the role of technology for some mitigation and adaptation options, reflecting specifically on how these contribute to tackling the risk of climate tipping points. The section also discusses the potential use of geoengineering, and in particular the use of Solar Radiation Modification technologies, as a response to the risks of crossing climate system tipping points.
Technologies needed for limiting warming to 1.5°C, with no or limited overshoot
Strategies that limit warming to 1.5°C with no or limited overshoot require accelerated near-term economic transformation, entailing rapid and deep emissions cuts worldwide. The ability to achieve these unprecedented levels of emissions reductions depends on technologies and the strength of reinforcing feedbacks. For example, the energy system is one of the key systems requiring transformation for a low-carbon world. Renewable power generation technologies including solar, wind and hydro technologies, are key for achieving that transition. The development of these technologies over the past decade led to a considerable decrease in the cost of energy from renewable sources and a significant scaling-up of renewable deployment and generation (IEA, 2021[13]). This new energy economy is the product of a virtuous cycle combining policy action and technology innovation, with momentum sustained by ever decreasing costs (IEA, 2021[13]). In every plausible low-carbon future where the crossing of climate system tipping points is avoided, these efforts need to be considerably accelerated, especially within the next decade (see section 3.2.for further detail).
The risk of climate tipping points also implies a key role for Carbon dioxide removal (CDR) technologies, as scenarios that limit warming to 1.5°C, with no or limited overshoot, all include at least some CDR. They serve three main purposes throughout the century: they help accelerating early deep emissions reductions, they balance out harder-to-abate sectors that continue to act as sources of residual emissions and they allow for reducing warming after peak temperature (Riahi et al., 2021[24]). It is important to note however that, since an overshoot could lead to the crossing of tipping points, the well-known argument that delayed emissions reductions can potentially be compensated by negative emissions during the latter part of the century is no longer valid.
CDR options in energy-system modelling pathways are mostly land-based biological CDR, including bioenergy with carbon capture and storage (BECCS), afforestation or soil-carbon sequestration and direct air CO2 capture and storage (DACCS) (M. Pathak, 2022[22]). While afforestation and soil-carbon sequestration have been practiced for decades to millennia (although not necessarily with the intention to remove carbon from the atmosphere), experience with BECCS and DACCS, whilst growing, is still limited (M. Pathak, 2022[22]). There remain today many legitimate concerns regarding BECCS, stemming primarily from its immense demand for land and resulting implications for land-use practices, trading-off available land for food production and impacting natural ecosystems and biodiversity (Creutzig et al., 2021[93]). It is argued that these risks may be best avoided by demand-side measures driving rapid decarbonisation in place of land-intensive carbon dioxide removal technologies (Creutzig et al., 2021[93]). Given that all scenarios in line with 1.5°C, with no or limited overshoot, include CDR, even when stringent demand-side measures are adopted, not employing CDR at all could result in the crossing of tipping points. The risks associated with BECCS can therefore no longer be considered in an isolated manner and need to be weighed against the risk of crossing tipping points. These potential trade-offs remain poorly understood and could greatly benefit from further research.
Knowledge transfer/sharing supporting transformational adaptation and M&E processes
Identifying technologies supporting climate change adaptation is a complex process that cuts across all sectors of the economy and at different scales. This section does not aim at providing a list of potential technologies, rather it focuses on key aspects identified in Section 3.3 and potential ways technology can harness their development. First, transformational adaptation approaches are still in their infancy. Supporting policy-makers in achieving transformational adaptation strategies may require both soft and hard technologies. For example, knowledge creation and transfer, a soft-technology, is key to informing decision-makers and ensuring transformational adaptation is possible. Boon et al., (2021[94]) argue that transformational adaptation may require the transformation of climate services in a process that simplifies and aggregates climate system knowledge, and then integrates it with knowledge about the physical, economic and social systems of cities and regions. The authors identify four knowledge requirements: “(1) system knowledge to identify the root causes [of climate risk] and solutions; (2) inspirational and cross-disciplinary knowledge to develop a long-term vision; (3) a clear climate message and guiding principles to mainstream the vision; and (4) design principles that are connected to the priorities and interests of the stakeholders.” (Boon et al., 2021[94]).
Scientific and modelling advances can also support adaptation approaches that are effective and in line with transformational adaptation, especially in the context of high-uncertainties associated with climate system tipping points. Palmer and Stevens, (2019[95]) argue that greater and more co-ordinated investments into climate modelling capabilities could reduce uncertainties in climate projections. While there are clear benefits from greater coordination and investments in climate modelling, the notion that simply increasing computational effort will necessarily reduce uncertainties of modelling output is disputed, particularly in relation to regional model responses (Stainforth and Calel, 2020[96]).
Geoengineering: the potential use of Solar Radiation Modification technologies
Solar geoengineering, and in particular, Solar Radiation Modification (SRM) approaches have been proposed as a potential strategy to reduce future climate impacts, as well as reducing the risk of crossing climate system tipping points (Irvine, Sriver and Keller, 2012[97]; Curry et al., 2014[98]; Heutel, Moreno-Cruz and Shayegh, 2016[99]). One well-known SRM approach consists of injecting sulphate aerosols in the stratosphere, thereby reducing the amount of solar radiation reaching the Earth’s surface. Such approaches have the potential to offset warming, even without a reduction in GHG emissions, however, their effectiveness and political feasibility of such approaches is debated (Barrett et al., 2014[100]; Irvine, Schäfer and Lawrence, 2014[101]).
In particular, there are concerns regarding risks associated with the use of SRM technologies. The latest IPCC report cautions that there remains little understanding of the potential of these technologies to actually reduce risk, and that they may also introduce novel risks to people and ecosystems (Pörtner et al., 2022[14]). The report highlights that SRM could result in substantial residual climate change by altering regional and global climate patterns (Ibid). In addition, because SRM does not change trends in emissions, climate impacts that are not directly related to temperature would not be avoided, such as ocean acidification under continued anthropogenic emissions. The report highlights there is high agreement in the literature that addressing climate change risks with SRM should only be a supplement to mitigation strategies, and should be used solely to reduce residual risks. The report also highlights considerable knowledge gaps, with low confidence in projected benefits of SRM as well as in risks to crop yields, economies, human health, or ecosystems. Finally, the report recognises that co-evolution of SRM governance and research provides a chance for responsibly developing SRM technologies, guarding against potential risks and harms relevant across a full range of scenarios. Other types of risks consist in the so-called “termination shock”, whereby a potential sudden stop of deployment of a particular SRM technology could lead to rapid and damaging rise in temperatures with potentially very dramatic impacts (Parker and Irvine, 2018[102]).
Despite the uncertainties and potential risks surrounding SRM, Heutel, Moreno-Cruz and Shayegh, (2016[99]) argue that, considering the risk of climate tipping points, solar geoengineering should be included as a policy option in an optimal climate policy strategy. This is because SRM approaches have the ability to reduce temperatures much faster than mitigation, and thus may reduce the risk of reaching a tipping point even if mitigation responses are insufficient (Heutel, Moreno-Cruz and Shayegh, 2016[99]). The authors, however, also argue against using such approaches after a tipping point has been reached. It has been highlighted that decision-making concerning SRM needs to take a risk-risk framework approach, where risks of SRM are analysed against other risks such as risks associated with e.g. climate change itself, emissions reductions, CDR or adaptation (Tyler Felgenhauer et al., 2022[103]) and that opportunities to increased investments in research for atmospheric climate interventions need to be rapidly ramped up (Silverlining, 2019[104])
All considered, given the limited level of research on geoengineering technologies, low confidence in their effectiveness and the considerable risks they are associated with, this report concludes that they are not today a feasible policy option for reducing the risk of crossing tipping points. Since mitigation strategies continue to face implementation challenges, in particular in terms of technological risks, scaling and costs, investments in technologies that can deliver 1.5°C through GHG mitigation need to be prioritised over more uncertain, and potentially riskier, geoengineering technologies.
References
[65] Agapiou, A. (2016), “Remote sensing heritage in a petabyte-scale: satellite data and heritage Earth Engine© applications”, International Journal of Digital Earth, Vol. 10/1, pp. 85-102, https://doi.org/10.1080/17538947.2016.1250829.
[67] Allis, E. et al. (2019), “The future of climate services”, No. 68, WMO Bulletin, World Meteorological Organization, Geneva, https://public.wmo.int/en/resources/bulletin/future-of-climate-services.
[34] Ara Begum, R. (2022), “Point of Departure and Key Concepts”, in H.-O. Pörtner, D. (ed.), Climate Change 2022: Impacts, Adaptation, and Vulnerability. Contribution of Working Group II to the Sixth Assessment Report of the Intergovernmental Panel on Climate Change, Cambridge University Press, In Press.
[62] Arias, P. et al. (2021), “Technical Summary”, in Masson-Delmotte, V. et al. (eds.), Climate Change 2021: The Physical Science Basis. Contribution of Working Group I to the Sixth Assessment Report of the Intergovernmental Panel on Climate Change, Cambridge University Press, Cambridge, United Kingdom and New York, NY, USA.
[100] Barrett, S. et al. (2014), “Climate engineering reconsidered”, Nature Climate Change, Vol. 4/7, pp. 527-529, https://doi.org/10.1038/nclimate2278.
[83] Bathiany, S., J. Hidding and M. Scheffer (2020), “Edge detection reveals abrupt and extreme climate events”, Journal of Climate, Vol. 33/15, pp. 6399-6421, https://doi.org/10.1175/jcli-d-19-0449.1.
[105] Bhave, A. et al. (2018), “Water Resource Planning Under Future Climate and Socioeconomic Uncertainty in the Cauvery River Basin in Karnataka, India”, Water Resources Research, Vol. 54/2, pp. 708-728, https://doi.org/10.1002/2017wr020970.
[52] Bloemen, P. et al. (2017), “Lessons learned from applying adaptation pathways in flood risk management and challenges for the further development of this approach”, Mitigation and Adaptation Strategies for Global Change, Vol. 23/7, pp. 1083-1108, https://doi.org/10.1007/s11027-017-9773-9.
[81] Boers, N. (2021), “Observation-based early-warning signals for a collapse of the Atlantic Meridional Overturning Circulation”, Nature Climate Change, Vol. 11/8, pp. 680-688, https://doi.org/10.1038/s41558-021-01097-4.
[94] Boon, E. et al. (2021), “Does Transformational Adaptation Require a Transformation of Climate Services?”, Frontiers in Climate, Vol. 3, https://doi.org/10.3389/fclim.2021.615291.
[80] Boulton, C. and T. Lenton (2015), “Slowing down of North Pacific climate variability and its implications for abrupt ecosystem change”, Proceedings of the National Academy of Sciences, Vol. 112/37, pp. 11496-11501, https://doi.org/10.1073/pnas.1501781112.
[87] Brovkin, V. et al. (2021), “Past abrupt changes, tipping points and cascading impacts in the Earth system”, Nature Geoscience, Vol. 14/8, pp. 550-558, https://doi.org/10.1038/s41561-021-00790-5.
[77] Bury, T., C. Bauch and M. Anand (2020), “Detecting and distinguishing tipping points using spectral early warning signals”, Journal of The Royal Society Interface, Vol. 17/170, p. 20200482, https://doi.org/10.1098/rsif.2020.0482.
[82] Bury, T. et al. (2021), “Deep learning for early warning signals of tipping points”, Proceedings of the National Academy of Sciences, Vol. 118/39, https://doi.org/10.1073/pnas.2106140118.
[26] Byers, E. (2022), AR6 Scenarios Database hosted by IIASA, International Institute for Applied Systems Analysis, https://doi.org/10.5281/zenodo.5886912.
[36] Cai, Y. et al. (2015), “Environmental tipping points significantly affect the cost-benefit assessment of climate policies”, Proceedings of the National Academy of Sciences of the United States of America, Vol. 112/15, pp. 4606-4611, https://doi.org/10.1073/PNAS.1503890112/SUPPL_FILE/PNAS.201503890SI.PDF.
[19] Canadell, J. et al. (2021), “Global Carbon and other Biogeochemical Cycles and Feedbacks”, in Masson-Delmotte, V. et al. (eds.), Climate Change 2021: The Physical Science Basis. Contribution of Working Group I to the Sixth Assessment Report of the Intergovernmental Panel on Climate Change, Cambridge University Press, Cambridge, United Kingdom and New York, NY, USA.
[59] CCC (2021), Progress in adapting to climate change: 2021 Report to Parliament.
[8] Chen, D. (2021), “Framing, Context, and Methods.”, in Masson-Delmotte, V. (ed.), Climate Change 2021: The Physical Science Basis. Contribution of Working Group I to the Sixth Assessment Report of the Intergovernmental Panel on Climate Change.
[72] CIF (2020), Strengthening Weather and Climate Information Serices: Highlights from PPCR Supported Projects, Climate Investment Funds, Washington, DC, https://www.climateinvestmentfunds.org/sites/cif_enc/files/knowledge-documents/ppcr_resilience_series_weather_and_climate_information_services.pdf.
[12] Climate Action Tracker (2021), Glasgow’s 2030 credibility gap: net zero’s lip service to climate action Wave of net zero emission goals not matched by action on the ground, https://climateactiontracker.org/publications/glasgows-2030-credibility-gap-net-zeros-lip-service-to-climate-action/ (accessed on 10 November 2021).
[21] Climate Action Traker (2015), 2.7°C is not enough – we can get lower, https://climateactiontracker.org/documents/44/CAT_2015-12-08_2.7degCNotEnough_CATUpdate.pdf (accessed on 15 June 2022).
[28] Copernicus EU (2022), Wildfire activity in the higher latitudes during spring and early summer.
[92] Cornforth, R., C. Petty and G. Walker (2021), “Supporting Climate-Resilient Planning at National and District Levels: A Pathway to Multi-stakeholder Decision-Making in Uganda”, in Climate Risk in Africa, Springer International Publishing, Cham, https://doi.org/10.1007/978-3-030-61160-6_8.
[93] Creutzig, F. et al. (2021), “Considering sustainability thresholds for BECCS in IPCC and biodiversity assessments”, GCB Bioenergy, Vol. 13/4, pp. 510-515, https://doi.org/10.1111/gcbb.12798.
[98] Curry, C. et al. (2014), “A multimodel examination of climate extremes in an idealized geoengineering experiment”, Journal of Geophysical Research: Atmospheres, Vol. 119/7, pp. 3900-3923, https://doi.org/10.1002/2013jd020648.
[58] Defra (2022), UK Climate Change Risk Assessment 2022.
[60] Dessai, S. et al. (2018), “Building narratives to characterise uncertainty in regional climate change through expert elicitation”, Environmental Research Letters, Vol. 13/7, p. 074005, https://doi.org/10.1088/1748-9326/aabcdd.
[39] Dietz, S. et al. (2021), “Economic impacts of tipping points in the climate system”, Proceedings of the National Academy of Sciences, Vol. 118/34, https://doi.org/10.1073/pnas.2103081118.
[75] Ditlevsen, P. and S. Johnsen (2010), “Tipping points: Early warning and wishful thinking”, Geophysical Research Letters, Vol. 37/19, https://doi.org/10.1029/2010gl044486.
[85] ESA (2021), “Remote Sensing Of Tipping Points In The Climate System”, webpage, https://climate.esa.int/en/news-events/remote-sensing-tipping-points-climate-system/ (accessed on 18 Oct 2021).
[64] Franzke, C. et al. (2022), “Perspectives on tipping points in integrated models of the natural and human Earth system: cascading effects and telecoupling”, Environmental Research Letters, Vol. 17/1, p. 015004, https://doi.org/10.1088/1748-9326/ac42fd.
[89] Geo-Wiki (n.d.), Risk Geo-Wiki, https://geo-wiki.org/branches/risk/ (accessed on 13 July 2022).
[79] Gsell, A. et al. (2016), “Evaluating early-warning indicators of critical transitions in natural aquatic ecosystems”, Proceedings of the National Academy of Sciences, Vol. 113/50, pp. E8089-E8095, https://doi.org/10.1073/pnas.1608242113.
[84] Guo, H., L. Zhang and L. Zhu (2015), “Earth observation big data for climate change research”, Advances in Climate Change Research, Vol. 6/2, pp. 108-117, https://doi.org/10.1016/j.accre.2015.09.007.
[44] H.-O. Pörtner, D. (ed.) (2022), Decision Making Options for Managing Risk, Cambridge University Press.
[45] Haasnoot, M. et al. (2019), “Generic adaptation pathways for coastal archetypes under uncertain sea-level rise”, Environmental Research Communications, Vol. 1/7, p. 071006, https://doi.org/10.1088/2515-7620/ab1871.
[63] Haasnoot, M. et al. (2013), “Dynamic adaptive policy pathways: A method for crafting robust decisions for a deeply uncertain world”, Global Environmental Change, Vol. 23/2, pp. 485-498, https://doi.org/10.1016/j.gloenvcha.2012.12.006.
[47] Heltberg, R., P. Siegel and S. Jorgensen (2009), “Addressing human vulnerability to climate change: Toward a ‘no-regrets’ approach”, Global Environmental Change, Vol. 19/1, pp. 89-99, https://doi.org/10.1016/j.gloenvcha.2008.11.003.
[91] Hemmerling, S. et al. (2019), “Elevating local knowledge through participatory modeling: active community engagement in restoration planning in coastal Louisiana”, Journal of Geographical Systems, Vol. 22/2, pp. 241-266, https://doi.org/10.1007/s10109-019-00313-2.
[99] Heutel, G., J. Moreno-Cruz and S. Shayegh (2016), “Climate tipping points and solar geoengineering”, Journal of Economic Behavior & Organization, Vol. 132, pp. 19-45, https://doi.org/10.1016/j.jebo.2016.07.002.
[68] Hewitt, C. et al. (2020), “Making society climate resilient: International progress under the Global Framework for Climate Services”, Bulletin of the American Meteorological Society, Vol. 101/2, pp. E237-E252, https://doi.org/10.1175/bams-d-18-0211.1.
[10] Howe, L. et al. (2019), “Acknowledging uncertainty impacts public acceptance of climate scientists’ predictions”, Nature Climate Change, Vol. 9/11, pp. 863-867, https://doi.org/10.1038/s41558-019-0587-5.
[13] IEA (2021), World Energy Outlook 2021, IEA, Paris, https://www.iea.org/reports/world-energy-outlook-2021 (accessed on 6 July 2022).
[33] IPCC (2022), “Summary for Policymakers”, in H.-O. Pörtner et al. (eds.), Climate Change 2022: Impacts, Adaptation, and Vulnerability. Contribution of Working Group II to the Sixth Assessment Report of the Intergovernmental Panel on Climate Change . In Press., Cambridge University Press.
[23] IPCC (2022), “Summary for Policymakers”, in P.R. Shukla et al. (eds.), Climate Change 2022: Mitigation of Climate Change. Contribution of Working Group III to the Sixth Assessment Report of the Intergovernmental Panel on Climate Change, Cambridge University Press, Cambridge, United Kingdom and New York, NY, USA.
[17] IPCC (2022), The evidence is clear: the time for action is now. We can halve emissions by 2030, https://www.ipcc.ch/2022/04/04/ipcc-ar6-wgiii-pressrelease/ (accessed on 15 August 2022).
[1] IPCC (2021), “Annex VII: Glossary”, in Matthews, J. et al. (eds.), n Climate Change 2021: The Physical Science Basis. Contribution of Working Group I to the Sixth Assessment Report of the Intergovernmental Panel on Climate Change, Cambridge University Press, Cambridge, United Kingdom and New York, NY, USA.
[16] IPCC (2021), “Summary for Policymakers”, in Masson-Delmotte, P. et al. (eds.), Climate Change 2021: The Physical Science Basis. Contribution of Working Group I to the Sixth Assessment Report of the Intergovernmental Panel on Climate Change, In Press.
[15] IPCC (2018), “Summary for Policymakers”, in Masson-Delmotte, V. et al. (eds.), Global Warming of 1.5°C. An IPCC Special Report on the impacts of global warming of 1.5°C above pre-industrial levels and related global greenhouse gas emission pathways, in the context of strengthening the global response to the threat of climate...., Intergovernmental Panel on Climate Change, Geneva.
[6] IRGC (2017), Introduction to the IRGC Risk Governance Framework, revised version, EPFL International Risk Governance Center, Lausanne, https://doi.org/10.5075/epfl-irgc-233739.
[101] Irvine, P., S. Schäfer and M. Lawrence (2014), “Solar radiation management could be a game changer”, Nature Climate Change, Vol. 4/10, pp. 842-842, https://doi.org/10.1038/nclimate2360.
[97] Irvine, P., R. Sriver and K. Keller (2012), “Tension between reducing sea-level rise and global warming through solar-radiation management”, Nature Climate Change, Vol. 2/2, pp. 97-100, https://doi.org/10.1038/nclimate1351.
[70] ITU (2020), Space Science for Achieving the Sustainable Development Goals, International Telecommunication Union, Geneva.
[41] Kates, R., W. Travis and T. Wilbanks (2012), “Transformational adaptation when incremental adaptations to climate change are insufficient”, Proceedings of the National Academy of Sciences, Vol. 109/19, pp. 7156-7161, https://doi.org/10.1073/pnas.1115521109.
[2] King, D. et al. (2015), CLIMATE CHANGE A RISK ASSESSMENT.
[4] Klinke, A. and O. Renn (2012), “Adaptive and integrative governance on risk and uncertainty”, Journal of Risk Research, Vol. 15/3, pp. 273-292, https://doi.org/10.1080/13669877.2011.636838.
[46] Lapola, D. et al. (2018), “Limiting the high impacts of Amazon forest dieback with no-regrets science and policy action”, Proceedings of the National Academy of Sciences, Vol. 115/46, pp. 11671-11679, https://doi.org/10.1073/pnas.1721770115.
[56] Leiter, T. (2021), “Do governments track the implementation of national climate change adaptation plans? An evidence-based global stocktake of monitoring and evaluation systems”, Environmental Science and Policy, Vol. 125, pp. 179-188, https://doi.org/10.1016/j.envsci.2021.08.017.
[76] Lenton, T. (2011), “Early warning of climate tipping points”, Nature Climate Change, Vol. 1/4, pp. 201-209, https://doi.org/10.1038/nclimate1143.
[86] Lenton, T. et al. (2019), “Climate tipping points — too risky to bet against”, Nature, Vol. 575/7784, pp. 592-595, https://doi.org/10.1038/d41586-019-03595-0.
[40] Loginova, J. and S. Batterbury (2019), “Incremental, transitional and transformational adaptation to climate change in resource extraction regions”, Global Sustainability, Vol. 2, https://doi.org/10.1017/sus.2019.14.
[37] Lontzek, T. et al. (2015), “Stochastic integrated assessment of climate tipping points indicates the need for strict climate policy”, https://doi.org/10.1038/NCLIMATE2570.
[31] Lovejoy, T. and C. Nobre (2019), “Amazon tipping point: Last chance for action”, Science Advances, Vol. 5/12, https://doi.org/10.1126/sciadv.aba2949.
[30] Lovejoy, T. and C. Nobre (2018), “Amazon Tipping Point”, Science Advances, Vol. 4/2, https://doi.org/10.1126/sciadv.aat2340.
[22] M. Pathak, R. (2022), “Technical Summary”, in P.R. Shukla et al. (eds.), Climate Change 2022: Mitigation of Climate Change. Contribution of Working Group III to the Sixth Assessment Report of the Intergovernmental Panel on Climate Change, Cambridge University Press, Cambridge, United Kingdom and New York, NY, USA.
[7] McKay, A. et al. (2022), “Exceeding 1.5°C global warming could trigger multiple climate tipping points”, Science, Vol. 377/6611, https://doi.org/10.1126/science.abn7950.
[90] Mechler, R. et al. (2018), “Supporting Climate Risk Management at Scale. Insights from the Zurich Flood Resilience Alliance Partnership Model Applied in Peru & Nepal”, in Loss and Damage from Climate Change, Climate Risk Management, Policy and Governance, Springer International Publishing, Cham, https://doi.org/10.1007/978-3-319-72026-5_17.
[35] Mechler, R. et al. (2020), “Loss and Damage and limits to adaptation: recent IPCC insights and implications for climate science and policy”, Sustainability Science, Vol. 15/4, pp. 1245-1251, https://doi.org/10.1007/s11625-020-00807-9.
[18] Meinshausen, M. et al. (2022), “Realization of Paris Agreement pledges may limit warming just below 2 °C”, Nature, Vol. 604/7905, pp. 304-309, https://doi.org/10.1038/s41586-022-04553-z.
[27] Miner, K. et al. (2022), “Permafrost carbon emissions in a changing Arctic”, Nature Reviews Earth & Environment 2022 3:1, Vol. 3/1, pp. 55-67, https://doi.org/10.1038/s43017-021-00230-3.
[9] Molina, T. and E. Abadal (2021), “The Evolution of Communicating the Uncertainty of Climate Change to Policymakers: A Study of IPCC Synthesis Reports”, Sustainability, Vol. 13/5, p. 2466, https://doi.org/10.3390/su13052466.
[32] Nepstad, D. et al. (2008), “Interactions among Amazon land use, forests and climate: prospects for a near-term forest tipping point”, Philosophical Transactions of the Royal Society B: Biological Sciences, Vol. 363/1498, pp. 1737-1746, https://doi.org/10.1098/rstb.2007.0036.
[51] New, M. et al. (2022), “Decision Making Options for Managing Risk”, in H.-O. Pörtner et al. (eds.), Climate Change 2022: Impacts, Adaptation, and Vulnerability. Contribution of Working Group II to the Sixth Assessment Report of the Intergovernmental Panel on Climate Change, Cambridge University Press.
[54] Noltze, M. et al. (2021), Monitoring, evaluation and learning for climate risk management, OECD Publishing, https://doi.org/10.1787/58665de0-en.
[73] O’Neill, B. et al. (2017), “The roads ahead: Narratives for shared socioeconomic pathways describing world futures in the 21st century”, Global Environmental Change, Vol. 42, pp. 169-180, https://doi.org/10.1016/j.gloenvcha.2015.01.004.
[57] OECD (2022), Measuring progress in implementing adaptation policies in the United Kingdom [forthcoming].
[5] OECD (2021), Managing Climate Risks, Facing up to Losses and Damages, OECD Publishing, Paris, https://doi.org/10.1787/55ea1cc9-en.
[53] OECD (2015), National Climate Change Adaptation: Emerging Practices in Monitoring and Evaluation, https://doi.org/10.1787/9789264229679-en.
[55] OECD (2023 (forthcoming)), Measuring progress in implementing national adaptation policies.
[48] O’Neill, B. (2022), “Key Risks Across Sectors and Regions”, in H.-O. Pörtner, D. (ed.), Climate Change 2022: Impacts, Adaptation, and Vulnerability. Contribution of Working Group II to the Sixth Assessment Report of the Intergovernmental Panel on Climate Change.
[42] P.R. Shukla, J. (ed.) (2022), Mitigation and development pathways in the near- to mid-term, Cambridge University Press, Cambridge, UK and New York, NY, USA, https://doi.org/10.1017/9781009157926.006.
[95] Palmer, T. and B. Stevens (2019), “The scientific challenge of understanding and estimating climate change”, Proceedings of the National Academy of Sciences, Vol. 116/49, pp. 24390-24395, https://doi.org/10.1073/pnas.1906691116.
[50] Paris Agreement (2015), 15 December 2015, United Nations Treaty Collection Certified True Copies (CTCs) of Multilateral Treaties Deposited with the Secretary-General Chapter XXVII.7.d, https://treaties.un.org/pages/ViewDetails.aspx?src=TREATY&mtdsg_no=XXVII-7-d&chapter=27 (accessed on 28 April 2020).
[102] Parker, A. and P. Irvine (2018), “The Risk of Termination Shock From Solar Geoengineering”, Earth’s Future, Vol. 6/3, pp. 456-467, https://doi.org/10.1002/2017ef000735.
[14] Pörtner, H. et al. (2022), “Technical Summary”, in H.-O. Pörtner et al. (eds.), Climate Change 2022: Impacts, Adaptation, and Vulnerability. Contribution of Working Group II to the Sixth Assessment Report of the Intergovernmental Panel on Climate Change, Cambridge University Press.
[66] Reichstein, M. et al. (2019), “Deep learning and process understanding for data-driven Earth system science”, Nature, Vol. 566/7743, pp. 195-204, https://doi.org/10.1038/s41586-019-0912-1.
[25] Riahi, K. (2022), Mitigation pathways compatible with long-term goals, Cambridge University Press, Cambridge, UK and New York, NY, USA, https://doi.org/10.1017/9781009157926.005.
[24] Riahi, K. et al. (2021), “Cost and attainability of meeting stringent climate targets without overshoot”, Nature Climate Change, Vol. 11/12, pp. 1063-1069, https://doi.org/10.1038/s41558-021-01215-2.
[20] Rogelj, J. et al. (2010), “Analysis of the Copenhagen Accord pledges and its global climatic impacts—a snapshot of dissonant ambitions”, Environmental Research Letters, Vol. 5/3, p. 034013, https://doi.org/10.1088/1748-9326/5/3/034013.
[78] Rosier, S. et al. (2021), “The tipping points and early warning indicators for Pine Island Glacier, West Antarctica”, The Cryosphere, Vol. 15/3, pp. 1501-1516, https://doi.org/10.5194/tc-15-1501-2021.
[43] Schipper, E. et al. (2022), “Climate Resilient Development Pathways”, in H.-O. Pörtner et al. (eds.), Climate Change 2022: Impacts, Adaptation, and Vulnerability. Contribution of Working Group II to the Sixth Assessment Report of the Intergovernmental Panel on Climate Change., Cambridge University Press.
[11] Shepherd, T. (2019), “Storyline approach to the construction of regional climate change information”, Proceedings of the Royal Society A: Mathematical, Physical and Engineering Sciences, Vol. 475/2225, p. 20190013, https://doi.org/10.1098/rspa.2019.0013.
[61] Shepherd, T. et al. (2018), “Storylines: an alternative approach to representing uncertainty in physical aspects of climate change”, Climatic Change, Vol. 151/3-4, pp. 555-571, https://doi.org/10.1007/s10584-018-2317-9.
[29] Sills, J. (ed.) (2020), “The dangers of Arctic zombie wildfires”, Science, Vol. 369/6508, pp. 1171-1171, https://doi.org/10.1126/science.abe1739.
[104] Silverlining (2019), Ensuring a Safe Climate A National - A National Imperative for Research in Climate Intervention and Earth System Prediction.
[96] Stainforth, D. and R. Calel (2020), “New priorities for climate science and climate economics in the 2020s”, Nature Communications, Vol. 11/1, https://doi.org/10.1038/s41467-020-16624-8.
[38] Steffen, W. et al. (2018), “Trajectories of the Earth System in the Anthropocene”, Proceedings of the National Academy of Sciences, Vol. 115/33, pp. 8252-8259, https://doi.org/10.1073/pnas.1810141115.
[3] Sutton, R. (2018), “ESD Ideas: a simple proposal to improve the contribution of IPCC WGI to the assessment and communication of climate change risks”, Earth System Dynamics, Vol. 9/4, pp. 1155-1158, https://doi.org/10.5194/esd-9-1155-2018.
[74] Swingedouw, D. et al. (2020), “Early warning from space for a few key tipping points in physical, biological, and social-ecological systems”, Surveys in Geophysics, Vol. 41/6, pp. 1237-1284, https://doi.org/10.1007/s10712-020-09604-6.
[103] Tyler Felgenhauer et al. (2022), “Solar Radiation Modification: A Risk-Risk Analysis”, https://www.c2g2.net/wp-content/uploads/202203-C2G-RR-Full.pdf (accessed on 16 August 2022).
[49] UNEP (2021), Adaptation Gap Report 2021.
[88] van Aalst, M., T. Cannon and I. Burton (2008), “Community level adaptation to climate change: The potential role of participatory community risk assessment”, Global Environmental Change, Vol. 18/1, pp. 165-179, https://doi.org/10.1016/j.gloenvcha.2007.06.002.
[71] WMO (2015), Valuing Weather and Climate: Economic Assessment of Meteorological and Hydrological Services, World Meteorological Organization, Geneva, https://library.wmo.int/doc_num.php?explnum_id=3314.
[69] WMO and GFCS (2019), “2019 State of climate services”, Working Paper, No. 1242, World Meteorological Organization, Geneva, https://library.wmo.int/doc_num.php?explnum_id=10089.
Notes
← 1. This report does not discuss or prejudge whether stakeholder engagement processes are effectively put in place, but acknowledges that these are also important factors of risk evaluation.
← 2. In this risk evaluation framework, how a risk is perceived is independent and prior to costs and/or cost-benefit considerations. However, even if the costs are considered, as reviewed in section 2.4, the costs of climate tipping points are projected to considerably outweigh mitigation costs.
← 3. A direct application of such an approach for the design of long-term adaptation pathways is given in Bhave et al (2018[105]).
← 4. Both soft and hard technology encompass scientifically ordered knowledge to develop goods or services that enable human adaptation. Hard technologies involve technological equipment such as example satellites, whereas soft technologies refers to technological knowledge, non-tangible material, administrative or organizational use.