This chapter deals with the genetics of Aedes aegypti, including genetic linkage to the physical map of the mosquito genome, its population genetics and its geographic distribution (phylogeography). Information is then provided on the genetics of insecticide susceptibility, the diverse modes of action and mechanisms of resistance to insecticides (metabolic, target-site, reduced penetration, behavioural avoidance). The last section focuses on the genetics of vector competence in Ae. aegypti, comprising the susceptibility of the mosquito populations to viruses and dengue virus in particular, the anatomic barriers to infection (midgut cells), the climatic factors affecting the infection susceptibility, the genetic variability and geographical variations in Ae. aegypti vector competence.
Safety Assessment of Transgenic Organisms in the Environment, Volume 8
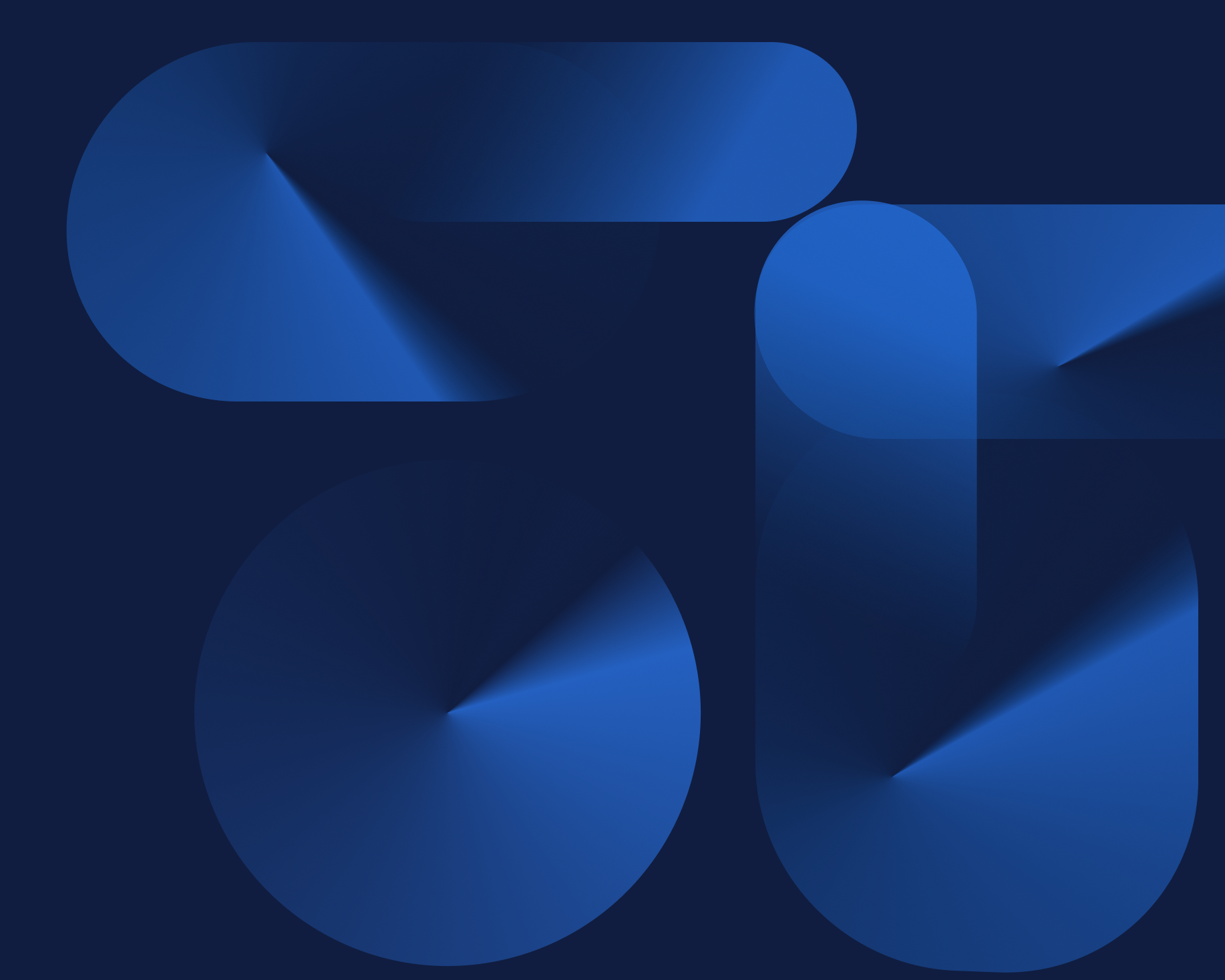
Chapter 3. Genetics of the mosquito Ae. aegypti
Abstract
Linkage map organisation of Ae. aegypti
Ae. aegypti was the first mosquito species for which a detailed genetic linkage map was constructed and linked to the physical map (Craig and Hickey, 1967; Munstermann and Craig, 1979). Sequence-tagged site (STS) markers were developed for two different strategies, both based on physical maps using fluorescence in-situ hybridisation (FISH). The first mapping strategy used cosmids (8 RFLP markers) and the second strategy used cDNAs (21) (Brown et al., 2001). Recently, a band-based approach was used to perform a physical mapping of the Ae. aegypti genome to its mitotic chromosomes (Sharakhova et al., 2011; Timoshevskiy et al., 2013). The mitotic chromosome complement of Ae. aegypti consists of three pairs of metacentric chromosomes (Rai, 1963) that are numbered 1 (smallest), 2 (largest) and 3 (intermediate) (McDonald and Rai, 1970). Ae. aegypti sex determination alleles have been linked to the smallest homomorphic autosome 1 (McClelland, 1962). The three chromosomes of Ae. aegypti have been subdivided into a total of 23 regions and 94 subdivisions based upon staining of early metaphase chromosomes using YOYO-1 iodide (Timoshevskiy et al., 2014) (Figure 3.1). In addition to 100 genetic markers and 183Mb of genomic sequence, a marker linked with sex determination (Severson et al., 2002) and 12 quantitative trait loci (QTL) associated with pathogen transmission (Severson et al., 1995; Bosio, Fulton and Salasek, 2000; Gomez‑Machorro, Bennett and Muñoz, 2004; Zhong et al., 2006) have been also anchored to the chromosomes (Timoshevskiy et al., 2014).
Although whole-genome sequencing has been undertaken (genome size of Ae. aegypti = 1.376 Gb), sequence compilation is still in progress due to the abundance of transposable elements (TEs) that cover approximately 50% of the Ae. aegypti genome (Nene et al., 2007; Severson and Behura, 2012; Timoshevskiy et al., 2014). TEs are extremely important to genome function and evolution (Arensburger et al., 2011) and may be key factors in mosquito genome plasticity. Low levels of polyteny resulting in poor quality of chromosome preparations add to the difficulty of using polytene chromosomes for physical mapping purposes in Ae. aegypti (Sharma et al., 1978; Campos, Andrade and Recco‑Pimentel, 2003). Furthermore, the abundance of TEs complicates FISH experiments which require the use of unlabelled repetitive DNA fractions to block non-specific hybridisation. A physical map for Ae. aegypti corresponding to 13.3% of the genome was developed using FISH markers on mitotic chromosomes (Severson et al., 1993), genetic linked map (RFLP cDNA-based map) (Brown et al., 1995, 2001; Sharakhova et al., 2011), and QTL (Severson et al., 1994, 1995; Bosio, Fulton and Salasek, 2000; Gomez-Machorro, Bennett and Muñoz, 2004; Zhong et al., 2006). More recently, a more detailed physical map of the mosquito was constructed and a total of 624 Mb covered approximately 45% of the Ae. aegypti genome (Timoshevskiy et al., 2014).
Though this mosquito has a high TE load including miniature inverted-repeat transposable elements (MITEs) and piRNA biogenesis genes, its genome has a low proportion of transposon-specific piRNAs (Biryukova and Ye, 2015). This is important in preserving overall genome stability because the small RNA pathway controls TE mobilisation and movement (Saito and Siomi, 2010; Senti and Brennecke, 2010). Unregulated movement of active elements or non-autonomous sequences can lead to insertional mutagenesis through the genome resulting in a decrease in genetic fitness. Arensburger (2011) also stated that the stability of the transposons in Ae. aegypti is the result of a low proportion of transposon-specific piRNAs.
Figure 3.1. A physical map of the Ae. aegypti genome

Note: Chromosome regions and subdivisions are indicated on the left side of the idiograms.
Source: Timoshevskiy, V.A. et al. (2013), “An integrated linkage, chromosome, and genome map for the yellow fever mosquito Aedes aegypti”, PLOS Neglected Tropical Diseases, Vol. 7, No. 2, pp. 2052; Timoshevskiy, V.A. et al. (2014), “Genomic composition and evolution of Aedes aegypti chromosomes revealed by the analysis of physically mapped supercontigs”, BMC Biology, Vol. 12, pp. 27.
RNA interference (RNAi) is an important anti-viral defence mechanism. Although the Ae. aegypti genome encodes RNAi component orthologs, however, most populations of this mosquito are readily infected by, and subsequently transmit, arboviruses (Nene et al., 2007; Arensburger et al., 2011).
Population genetics and phylogeography of Ae. aegypti
The two Ae. aegypti subspecies: Ae. aegypti formosus, a wild mosquito apparently limited to sub-Saharan Africa, and Ae. aegypti aegypti, found globally in tropical and subtropical regions typically in association with humans (Moore et al., 2013), are described with their characteristics under the Classification (Taxonomy) section in Chapter 1. In addition, the Systematics section reports on the existence of two principal clades of Ae. aegypti collections worldwide.
In Mexico, local patterns of gene flow among Ae. aegypti populations were assessed using random amplified polymorphic DNA (RAPD) markers. Large genetic distances were observed, suggesting reduced gene flow among the mosquitoes (García-Franco et al., 2002; Gorrochotegui-Escalante et al., 2002; Muñoz et al., 2013a). The populations are panmictic along the Pacific coast, isolated by distance in northeast Mexico, and exhibit moderate gene flow across the Yucatan peninsula (Muñoz et al., 2013a). In the southern Pacific coast region reduced gene flow may result from sampling at altitudes greater than 1 500 m, which is close to the altitudinal limit for Ae. aegypti in Mexico (Lozano-Fuentes et al., 2009; Navarro et al., 2010), the mosquito being unable to survive at altitudes greater than 2 000 m (Lozano-Fuentes et al., 2012).
Sequence variation in the mitochondrial NADH dehydrogenase subunit 4 (ND4) gene, has been used to describe patterns of gene flow among Ae. aegypti s.l. collections within and among countries outside Africa (Gorrochotegui-Escalante et al., 2000, 2002; Bosio et al., 2005; Costa-da-Silva, 2005; Herrera et al., 2006; Bracco et al., 2007; Ribeiro et al., 2007; Paduan and Ribolla, 2008; Paupy et al., 2008; Urdaneta-Marquez et al., 2008; Dueñas et al., 2009; Hlaing et al., 2009; Lima and Scarpassa, 2009; Lozano-Fuentes et al., 2009; Paupy et al., 2012; Muñoz, 2013a; Moore et al., 2013). In Mexico, novel ND4 haplotypes were discovered and used to assess the amount of gene flow among breeding sites and to possibly predict the degree to which dengue virus (DENV) is transferred among sites (Gorrochotegui-Escalante et al., 2000, 2002; García-Franco et al., 2002).
To date 96 novel ND4 haplotypes have been discovered and three phylogenetic patterns have been consistently noted: either mtDNA haplotypes were distributed as two well‑supported clades (Gorrochotegui-Escalante et al., 2000; Bosio et al., 2005; Lima and Scarpassa, 2009), or as a basal group similar to outgroup subspecies from which a second derived clade arises (Gorrochotegui-Escalante et al., 2002; Bracco et al., 2007; Paduan and Ribolla, 2008; Dueñas et al., 2009; Hlaing et al., 2009; Lozano-Fuentes et al., 2009; Paupy et al., 2012; Muñoz et al., 2013a). The broad distribution of specific haplotypes in Venezuela (Urdaneta-Marquez et al., 2008), Brazil (Bracco et al., 2007; Paduan and Ribolla, 2008; Lima and Scarpassa, 2009), Guatemala (Bracco et al., 2007), and Peru (Costa-da-Silva, 2005) demonstrates efficient mosquito dispersion in Central and South America.
Control practices are implicated as a major cause of genetic drift in Ae. aegypti. This was the conclusion of a study investigating 19 Ae. aegypti collections in Thailand, from Chiang Mai in the north to Songkhla province in the south (Bosio et al., 2005). That study found seven mitochondrial ND4 haplotypes, no evidence of isolation by distance, and low gene flow estimates among collections. They also concluded that these patterns are consistent with genetic drift arising from vector control efforts. Furthermore, polymorphisms were examined at 10 isoenzyme loci among 15 Ae. aegypti collections from Chiang Mai (Mousson et al., 2002). Low gene flow was also detected among these collections. These authors also concluded that this pattern was related to insecticide treatments. Additional studies further demonstrate the contribution of insecticide exposure to genetic drift in Martinique (Marcombe et al., 2009, 2012, 2013), Phnom Penh (Paupy et al., 2004) and French Guiana (Failloux et al., 2002). More on natural factors and human activities affecting gene flow or distribution is given under Chapter 4.
In summary, because Ae. aegypti is the primary global vector of severe viral diseases to humans (see Annexes A and B), it is crucial to study its population genetics in order to develop strategies to control the dispersion of the mosquito. Studies over the past 50 years have shown large differences among global populations of this species. Past studies based on morphological polymorphisms and allozymes were recently completed by the use of molecular genetic markers (including microsatellites and mitochondrial markers). Phylogenetic analyses consistently resolved two clades. In addition, phylogenetic analyses showed that populations of Ae. aegypti outside Africa consist of mosquitoes arising from two ancestral clades; one is basal and primarily associated with West Africa while the second arises from the first and contains primarily mosquitoes from East Africa. Across these many studies on population genetics, and those based on the distribution of the mosquito haplotypes around the world, it can be suggested that mosquito dispersion is very efficient, most likely due to commercial transportation and human movements.
Genetics of insecticide susceptibility and development of insecticide resistance
Resistance to insecticides
According to the World Health Organization (WHO, 2012a, 2012b), insecticide resistance is defined as the ability of an insect to withstand the effects of an insecticide by becoming resistant to its toxic effects by means of mutation and natural selection. Appropriate tools (biological, biochemical and/or molecular) are needed to identify the mechanisms involved in developing resistance and to conduct surveillance at individual and/or population levels.
Such resistance has been observed in more than 500 insect species worldwide, including more than 20 Aedes species (Diptera: Culicidae). Over 400 scientific reports worldwide document insecticide resistance in Ae. aegypti.
The large use of insecticides, and the resultant selection pressure on insect populations, has led to widespread resistance to all classes of insecticides among many invertebrate pests, making control difficult. Frequent applications of the same insecticide will select for those individuals in a population that are able to survive the recommended rates of the compounds owing to a genetically-fixed difference. Over time, this selection pressure will lead to a resistant population becoming established. In such cases, other compounds within the same class of chemistry are most often also affected; for instance, resistance to one pyrethroid type usually confers resistance across the whole group of pyrethroids, a phenomenon known as cross-resistance. Sometimes, depending on the nature of the resistance mechanism, multi-resistance can occur between different chemical classes, for example organophosphates and carbamates. The frequent treatments of crops with similar synthetic insecticides may also indirectly affect the susceptibility of insects of public health importance, with insect vectors additionally exposed when in the vicinity of agricultural sprays (Brogdon and McAllister, 1998; Hemingway and Ranson, 2000; Liu et al., 2006).
The portfolio of insecticides available for management of arthropod vectors (PAHO, 1994; WHOPES, 2005) is very limited and unlikely to increase dramatically in the near future. Development of resistance to commonly-used insecticides is therefore a serious threat to human ability to combat mosquito-borne diseases. Insecticide susceptibility must be viewed as a valuable “natural resource” at risk for being depleted. This underscores the critical importance of monitoring insecticide resistance through development and implementation of relevant management schemes. More information is contained in the section on “Prevention and management of insecticide resistance” of Annex A.
Insecticides, mode of action and resistance mechanisms
Vector control programmes include activities to control both immature and adult stages of Ae. aegypti. Chemical or biological larviciding and physical source reduction of container habitats are intended to control larvae, but house-to-house larval control is too laborious for sustainable implementation by vector control programmes or community participation (Reiter and Gubler, 1997; WHO, 2009; Horstick et al., 2010). During dengue outbreaks, outdoor and indoor spraying of insecticides is used to kill adults (WHO, 2009; Esu et al., 2010). Control measures are explored with more details in Annex A.
The four chemical classes of insecticides (organochlorides, organophosphates, carbamates, pyrethroids) used for larvae and adult mosquito control have their biochemical target sites in the insect central nervous system, which makes them fast-acting killing agents. They act on only two different molecular target sites in the central nervous system, leading the insect to over-excitation and death. Organophosphates and carbamates both inhibit acetylcholinesterase (AChE), an enzyme of crucial importance in terminating nerve impulses by cleaving the natural neurotransmitter acetylcholine (Eto, 1974). In contrast, synthetic pyrethroids (and DDT, representing the organochlorides) modulate voltage-gated sodium channels, resulting in rapid knockdown properties (Khambay, 2002). It is important to note that these four chemical classes address only two different modes of action, so there is much less target-site diversity involved in the control of adult mosquitoes compared with the agricultural sector, which can rely on many more modes of action to date (Nauen and Bretschneider, 2002; Nauen, 2006). Insect growth regulator (IGR), pyriproxyfen is a juvenile hormone analogue that can be considered as an alternative to conventional insecticides because of its specific activity against immature insects, low persistence in the environment and virtually non-toxic to mammals (Madhu and Vijayan, 2009).
The various mechanisms that enable insects to resist the action of insecticides can be grouped into four distinct categories as follows: metabolic resistance, target-site resistance, reduced penetration, and behavioural avoidance.
Metabolic resistance
Metabolic resistance is the most common resistance mechanism that occurs in insects. This mechanism is based on the enzyme systems, which all insects possess to help them to detoxify naturally-occurring xenobiotics and insecticides. It is commonly accepted that insect detoxification systems derived from the plant-insect evolutionary arms race, and several insect detoxification enzymes have been associated with the detoxification of plant toxins and all types of chemicals, including insecticides (Despres, David and Gallet, 2007). Over-expression of enzymes capable of detoxifying insecticides or amino acid substitutions within these enzymes, which alter the affinity of the enzyme for the insecticide, can result in high levels of insecticide resistance (Hemingway et al., 2004; Flores et al., 2005, 2006). Increased expression of the genes encoding the major xenobiotic metabolising enzymes is the most common cause of insecticide resistance in mosquitoes. Over-expression of detoxifying enzymes can occur as the result of gene amplification (e.g. duplication) or due to changes in either transacting regulator elements or the promoter region of the gene (Guillemaud et al., 1997; Hemingway and Ranson, 2000; Hawkes and Hemingway, 2002). The consequence is a significant increase in enzyme production in resistant insects that enables them to metabolise or degrade insecticides before they are able to exert a toxic effect.
Three enzyme families (with a variable number of gene members), the cytochrome P450 monooxygenases (P450s), glutathione transferases (GST), and carboxyl/cholinesterases (CCE) are implicated in insecticide metabolism. Each of these catalyses a wide range of detoxification reactions. They are the primary enzymatic defence against xenobiotics, are responsible for the removal of many by-products of metabolism, play essential roles in multiple biosynthetic pathways and are involved in chemical communication (Feyereisen, 2005; Oakeshott et al., 2005; Ranson and Hemingway, 2005). Some individual enzymes also have structural roles instead of, or in addition to, their catalytic activity. This diversity in the function of each enzyme family is accomplished by a mixture of highly specialised enzymes, often with specific substrates and strictly regulated expression profiles, and more generalist, ubiquitously expressed enzymes. Many insect species show an amazing diversity of detoxification enzymes. As insect genomes have been sequenced, and the detoxification genes annotated, it has become apparent that these detoxification gene families are very rapidly evolving and each insect has a unique complement of detoxification genes, with very few orthologs across insect species (Ranson et al., 2002; Claudianos et al., 2006). The rapid expansion and diversification of detoxification genes likely facilitated the adaptation of insects to their particular ecological niches, and, on a more recent evolutionary timescale, has enabled them to survive various man-made xenobiotics, including insecticides. A small subset of the detoxification genes has been previously described in Ae. aegypti (Sieglaff, Duncan and Brown, 2005; David et al., 2006; Lumjuan et al., 2007).
Target-site resistance
Target-site resistance is the second most common mechanism of resistance to insecticides encountered in insects. Insecticides (e.g. organophosphates, carbamates, DDT and pyrethroids) generally act at a specific site within the insect, typically within the nervous system. The site of action can be modified in resistant insect strains such that the insecticide no longer binds effectively.
Reduced sensitivity of the target receptors to insecticide results from non-silent point mutations in the gene encoding the protein constituting the target site. For example, the target site for organophosphate and carbamate insecticides is AChE in the nerve cell synapses. Several mutations in the AChE gene have been found in insects (Fournier, 2005), which result in reduced sensitivity to inhibition of the enzyme by these insecticides (Weill et al., 2003).
Alterations in the target site that cause resistance to pyrethroids and DDT are often referred to as knockdown resistance (kdr), in reference to the ability of insects with relevant alleles to withstand prolonged exposure to insecticides without being ‘knocked-down’. The kdr is conferred principally by non-synonymous mutations in the voltage-gated sodium channel gene that reduce insecticide binding to this channel in the insect nerve sheath, thereby preventing the loss of co‑ordinated activity and paralysis in the insect (Soderlund and Knipple, 2003; Davies et al., 2007; Rinkevich, Du and Dong, 2013).
Worldwide, numerous kdr-conferring voltage-gated sodium channel allele mutations (e.g. S989P; I1,011M; I1,011V; V1,016I; V1,016G; F1,534C; and D1,794Y) have been described in Ae. aegypti (Vontas et al., 2012; Rinkevich, Du and Dong, 2013). Multiple kdr mutations had been reported from Mexico, the Caribbean, and Central America (Saavedra‑Rodriguez et al., 2007). Subsequent studies reported the presence of kdr conferring mutations in Ae. aegypti collections from Brazil (Martins et al., 2009a, 2009b; Lima et al., 2011; Belinato, Martin and Valle, 2012), Mexico (Ponce-Garcia et al., 2009; Siller et al., 2011; Aponte et al., 2013) and the Caribbean (Marcombe et al., 2009, 2012, 2013; Harris, Rajatileka and Ranson, 2010; Bariami et al., 2012; McAllister, Godsey and Scott, 2012; Maestre-Serrano et al., 2014; Alvarez et al., 2015).
Reduced penetration
Reduced penetration (and behavioural resistance by reduced penetration) occurs when insects develop a heritable mechanism(s) that reduces or prevents the entry of a toxin into the insect’s body. Modifications in the cuticle or digestive tract linings that prevent or slow the penetration/absorption of insecticides can be found in some resistant insects. This resistance mechanism is non-specific and can affect the effectiveness of a broad range of insecticides. Reduced uptake of insecticide, often referred to as cuticular resistance, is frequently described as a minor resistance mechanism. Certainly, for pests where the major route of insecticide delivery is via ingestion, this is likely to be the case. However, for dengue control, where insecticides are typically applied spatially or on wall surfaces, uptake of insecticides is primarily through the appendages. An increase in the thickness of the tarsal cuticle, or a reduction in its permeability to lipophilic insecticides, could have a major impact on the bioavailability of an insecticide in vivo.
Reduced cuticle penetration is the least understood resistance mechanism. Though it may have a primary role in resistance (Valles, Dong and Brenner, 2000; Ahmad, Denholm and Bromilow, 2006; Puinean et al., 2010), it more often acts in combination with the other mechanism(s).
Behavioural avoidance
Insecticide resistance in mosquitoes may also be conferred by behavioural changes in response to prolonged exposure to an insecticide. Behavioural avoidance does not have the same importance as physiological resistance but may be considered to be a contributing factor, leading to the avoidance of lethal doses of an insecticide (Chandre et al., 2000; Grieco et al., 2007). This type of response can be further divided into direct contact excitation (sometimes referred to as “irritancy”), and non-contact spatial repellency when insects move away from the insecticide-treated area before making direct contact (Chareonviriyaphap et al., 1997; Grieco et al., 2007).
To better approximate insect behaviour in natural field settings, numerous experiments have been made over many decades using specially constructed experimental huts (Smith, 1965; Rozendaal et al., 1989; Roberts and Alecrim, 1991; Bangs, 1999; Grieco et al., 2000, 2007; Polsomboon et al., 2008; Malaithong et al., 2010). Most experimental hut studies have been conducted to observe the behaviour of Anopheles mosquitoes; however, Grieco et al. (2007) successfully demonstrated that chemical actions could be observed in experimental huts using Ae. aegypti as a model system. The results obtained from both laboratory and field studies can help facilitate the choice of the most effective chemicals and measures to control house-frequenting adult mosquitoes.
Conclusion on resistance to insecticides
Insecticide resistance develops in an insect population when individuals carrying genes that allow them to survive exposure to the insecticide survive, mate and pass these genes onto the next generation. Thus, any activities that control the individuals with the resistance trait will delay the spread of the resistance genes in the population. Some elements on prevention and management of insecticide resistance are given in Annex A.
Genetics of vector competence in Ae. aegypti
Vector competence, a key factor
The vector competence (VC) is defined as the intrinsic permissiveness of an arthropod vector for infection, dissemination and transmission of a pathogen (Black et al., 2002; Dickson et al., 2014). The full competence of a vector is determined not only by its ability to become infected, but also by its ability to transmit a pathogen.
VC in Ae. aegypti is an element of primary importance to consider because of this mosquito’s public health impact as main potential transmitter of dengue, yellow fever, Zika and chikungunya viruses. In a few restricted areas, Ae. aegypti is also a vector of Wuchereria bancrofti and Brugia malayi, both of which cause lymphatic filariasis or elephantiasis (Service, 2012; Powell and Tabachnick, 2013)
Consequently, Ae. aegypti has been the subject of numerous vector competence and population genetic studies (Aitken, Downs and Shope, 1977; Gubler et al., 1979; Tabachnick and Powell, 1979; Rosen et al., 1985; Tabachnick et al., 1985; Tardieux et al., 1990; Miller and Mitchell, 1991; Apostol, Reiter and Miller, 1996; Bosio and Beaty, 1998; Vazeille-Falcoz et al., 1999; Bosio, Fulton and Salasek, 2000; Bennett et al., 2002b; Gorrochotegui-Escalante et al., 2002; Mercado-Curiel, Black and Muñoz, 2008; Lozano‑Fuentes et al., 2009; Sylla et al., 2009; Lambrechts, 2011; Lambrechts et al., 2011; Guo et al., 2013; Muñoz et al., 2013b; Chepkorir et al., 2014; Diagne et al., 2014; Dickson et al., 2014; Gonçalves et al., 2014; Vega-Rúa et al., 2014).
Susceptibility of Ae. aegypti populations to viruses
Infection with dengue virus
Ae. aegypti becomes infected with a viral disease, dengue for example, when the mosquito bites and acquires a blood meal from a dengue virus (DENV)-infected human, the primary host of the virus. The mosquito infection depends on factors such as DENV virulence, physical barriers and innate immunity that can confer resistance or susceptibility of an Ae. aegypti population to viruses.
The relationships between DENV and its arthropod vector Ae. aegypti are crucial, and an analysis of host cell responses to flavivirus infection of mosquito vectors is very important for understanding the maintenance and transmission of the disease.1 Mosquito populations differ in their susceptibility to flavivirus development (i.e. VC), reflecting the different barriers encountered by the virus from its entry into the mosquito to its release in the saliva. Factors such as specific mosquito epithelial cells receptors, as well as differential viral replication in the mosquito, are critical for VC - as are other genes as exhibited by quantitative trait loci (QTL) studies (Gomez-Machorro, Bennett and Muñoz, 2004).
Three Ae. aegypti strains with different susceptibilities to DENV infection have been reported (namely DS3, DMEB and IBO-11), and these have been used to study whether midgut cell receptors for DENV may be markers for VC (Bennett and Beaty, 2005). Ae. aegypti susceptibility to DENV, attributable to multiple genetic factors, is found to be usually very high, particularly against DENV-2,2 as elicited by DS3 and DMEB strains.
It has been observed that the three strains showed a difference in the degree of infection of their midgut (MG) cells, depending on their susceptibility to DENV. For example, the IBO‑11 strain expressed almost no infection remaining after 26 days post-infection. DMEB strain showed increase in infection up to 26 hours in all the three MG regions, having the maximal virus accumulation in the posterior MG which then diminished by 14 days post-infection, compared to the other susceptible strain DS3 that has maximal virus accumulation in anterior MG at 14 days post-infection. These results also display a statistically-significant MG infection increase from the first five hours post-infection to 26 hours in DS3 and DMEB strains (p < 0.05). Moreover, IBO-11 strain exhibited a significant decrease (p < 0.05) of MG infection from 5 to 336 hours post-infection. Virus infection of IBO-11 strain was almost completely abolished (p < 0.05) from 13 to 336 hours post-infection (Mercado-Curiel, Black and Muñoz, 2008). The susceptibility, resistance and refractoriness depend on multiple genetic factors (Miller and Mitchell, 1991).
Anatomic barriers to infection (midgut cells)
The VC for arboviruses is associated with a number of anatomic barriers to productive vector infection. These include a midgut infection barrier (MIB), a midgut escape barrier (MEB) and a salivary gland barrier (Black et al., 2002). In potential vectors provided with an MIB, a virus cannot infect and/or replicate in the mosquito MG cells. This may be due to a lack of specific cell surface receptors for the virus or to MG cells being non-permissive for infection with the virus (Mercado-Curiel, Black and Muñoz, 2008). Potential vectors provided with an MEB may allow virus replication in the MG, even to high titres (concentrations), but the virus is then unable to exit the MG to cause a disseminated infection. The VC for flaviviruses in Ae. aegypti is thought to be controlled by at least two genes or sets of genes, one controlling the MIB and the other controlling the MEB (Miller and Mitchell, 1991; Bosio and Beaty, 1998; Bosio, Fulton and Salasek, 2000). A study by Bennett et al. (2002b) also concluded that these barriers are probably major determinants of VC to DENV in nature and during experimental infections.
Bosio and Beaty (1998) proposed a significant additive genetic effect in MIB and demonstrated that the DENV titre in the mosquito MG and head did not correlate with the rate of infection. They also showed that the heritability for virus titres in tissues (MG or head) were almost identical in different strains of Ae. aegypti formosus and showed that the amount of virus in the MG did not determine if the virus was disseminated, which hypothetically may be due to the presence or absence of DENV receptors (in the MG, in particular).
Barriers to infection can vary widely in prevalence among Ae. aegypti populations, leading to large intraspecific variation of Ae. aegypti VC that may influence the epidemiology of DENV and other flaviviruses (Black et al., 2002).
Climatic factors affecting the infection susceptibility
Susceptibility of Ae. aegypti mosquito to DENV varies geographically and can be influenced by climatic factors such as temperature, which affect the incidence, seasonality and distribution of vector-borne diseases.
The VC has shown to be affected by temperature, which impacts biological processes of mosquitoes including their interaction with viruses (Watts et al., 1987; Lambrechts et al., 2011). Chepkorir et al. (2014) demonstrated a significantly higher infection rate at high temperatures for mosquitoes collected in Nairobi and Kilifi (Kenya), which is consistent with previous results (Watts et al., 1987). The 2014 study showed that the Nairobi Ae. aegypti population is a relatively inefficient vector for DENV-2 compared to that from Kilifi with the former showing high infection, but low dissemination rates in low- and high-temperature settings. These results also suggested a weak MIB and a strong MEB for the Nairobi population, and a moderate MIB but weak MEB for the Kilifi population.
Genetic variability and geographical variations in Ae. aegypti vector competence
A number of genetic studies on VC were conducted worldwide, which demonstrated a great VC variability. The results of some of these works performed in different countries are presented hereinafter. All in all, they indicate that vector control strategies should be adapted to the available data for each region. Further analysis should be conducted to better understand the reasons for this large variability in VC and how these parameters correlate with epidemiological findings (Gonçalves et al., 2014).
Ae. aegypti populations exhibit considerable genetic variability in VC for flaviviruses, including DENV-2 viruses. The range of VCs shown suggests that the ability to overcome the MIB and MEB to transmit DENV-2 JAM1409 is a quantitative trait with multiple genes that likely condition VC and collectively determine the infection rates of mosquito populations. This theory has been studied using crosses of susceptible and refractory mosquito lines (Miller and Mitchell, 1991; Bosio and Beaty, 1998).
Significant genetic variation in Ae. aegypti on a smaller scale has been demonstrated in Puerto Rico (Apostol, Reiter and Miller, 1996) and in Mexico (Gorrochotegui-Escalante et al., 2000). Subsequently, the potential variation in VC on a regional geographic scale was addressed in Mexico. The major aim of this research was to determine if genetic variability in Ae. aegypti populations conditions the incidence and severity of dengue fever and dengue haemorrhagic fever outbreaks. Such study can help identify genetic biomarkers for mosquito populations that pose undue risk for severe diseases and allow control programmes to focus their resources on areas at greatest risk.
Several studies have shown that Ae. aegypti has a continuous variation in its competence to transmit flavivirus (Bennett et al., 2002a; Black et al., 2002; Severson et al., 2004; Gorrochotegui-Escalante et al., 2005). Ae. aegypti from 24 collections in Mexico and the United States were challenged orally with DENV-2 JAM1409, and the VC of the populations ranged from 24% to 83%. In general, the Ae. aegypti collections from throughout Mexico exhibited considerable variability in VC, and collections from the Yucatan Peninsula were generally more competent than those from other geographic regions (Bennett et al., 2002b). Lozano-Fuentes et al. (2009) showed that the Neovolcanic Axis (NVA) in Mexico is a natural barrier to Ae. aegypti VC for DENV, as a much lower VC (20%) prevails for mosquito populations from south of the NVA compared to mosquitoes collected from north of the NVA (55%).
Ae. aegypti populations from Belo Horizonte, Brazil, exhibited wide variation in VC to transmit dengue. Most Brazilian states are infested with Ae. aegypti and are consequently at risk of dengue transmission (Figueiredo et al., 2008). Moncayo et al. (2004) studied populations from various geographical locations and showed that Ae. aegypti from Galveston, Texas (United States) were more susceptible than those from Bolivia but were less susceptible than mosquitoes from Thailand. This concurred with the observations made by Bennett et al. (2002b) on Ae. aegypti collected from various locations in Mexico and by Chepkorir et al. (2014) on populations from two different Kenyan sites, that all differed significantly in their MG susceptibility to infection.
The VC studies on Ae. aegypti from West Africa have shown that mosquitoes are more refractory for both DENV (Tabachnick et al., 1985; Bosio and Beaty, 1998) and yellow fever virus (YFV) (Tabachnick et al., 1985; Miller and Mitchell, 1991) compared to Ae. aegypti collected from the Americas or Asia (Diallo et al., 2005, 2008).
In studies directly comparing collections within Senegal, wide variation in VC was shown for both high-passage (Sylla et al., 2009) and low-passage field isolates of DENV-2 (Diallo et al., 2005, 2008). Especially, sylvatic collections from south-eastern Senegal were more refractory than other collections from throughout the country. However, it should be noted that the study by Sylla et al. (2009) only examined the highly passaged DENV-2 Jam1409 isolate (reinforcing the importance of using strains circulating in the geographic area of study). Previous studies demonstrated that geographically-distinct collections of Ae. aegypti from Senegal are genetically diverse and documented the great variability in VC for both DENV-2 and YFV across the country. The northwest-southeast decline in the susceptibility to YFV BA-55 is very similar to that seen with DENV-2 JAM1409 (Huber et al., 2008; Sylla et al., 2009).
Furthermore, it has been demonstrated that VC of Ae. aegypti for DENV is dependent on the interactions between the mosquito strain and virus genotype in natural collections (Lambrechts et al., 2009). Using viruses and vectors that are geographically proximate and genetically diverse is important in order to make strong conclusions about VC between collections. Assessing VC in Ae. aegypti with a viral isolate collected in proximity seems to be the most informative approach (Lambrechts et al., 2009). Diallo et al. (2005, 2008) followed this process by examining the VC of Ae. aegypti from Senegal with multiple local isolates of DENV-2. The 2008 study reported low levels of MG infection (0.0–26.3%) and variable disseminated infection (0‑100%) in six collections from Senegal regardless of geographic location. Both studies demonstrated variability in infection rates based on the isolate of DENV-2 and the collection site, confirming the local adaptation between the virus and the mosquito vector. They also showed that although collections of sylvatic Ae. aegypti presented lower infection rates than sylvatic Aedes from other species, some sylvatic Ae. aegypti mosquitos developed nevertheless a disseminated infection.
Similarly, Lambrechts et al. (2009) demonstrated that differences in VC among three Ae. aegypti collections from Thailand infected with three genotypes of DENV-1 was a result of mosquito and virus genotypes interactions. Dickson et al. (2014) also highlighted interactions between mosquito and virus genotypes in sylvatic Ae. aegypti, and with YFV in West Africa. Overall, VC was dependent upon both viral and vector strains. Importantly, and contrary to previous studies, the study by Dickson et al. (2014) reported that sylvatic collections of Ae. aegypti showed high levels of disseminated infection for local isolates of both DENV-2 and YFV.
Recently, VC of Ae. aegypti for chikungunya virus (CHIKV) has been investigated and it was found that Ae. aegypti populations from Cape Verde and Kedougou (Senegal) were competent for CHIKV, but Ae. aegypti from Dakar (Senegal) presented a low susceptibility to the virus. The virus strains belonging to the West African lineage were the only ones disseminated by the domestic population of Ae. aegypti from Dakar and transmitted by those from Cape Verde (Diagne et al., 2014). And as previously demonstrated in Kerala, India (Kumar et al., 2012), it has been also observed that Ae. albopictus was a better vector for this virus (CHIKV) than Ae. aegypti (Diagne et al., 2014).
In addition to DENV, CHIKV and YFV, Ae. aegypti is also a vector for Zika virus (ZIKV), a single-stranded RNA virus belonging to the Flaviviridae family (Faye et al., 2014; Abushouk, Negida and Ahmed, 2016). More details on ZIKV and related infection are given in Annex B, section “Virus infection vectored by mosquitoes”. The VC for ZIKV in Ae. aegypti is variable, depending on the source of the mosquitoes and the virus strain. Ae. aegypti collected from French Polynesia displayed high ZIKV infection rate, but late ability to transmit the virus (Richard, Paoaafaite and Cao-Lormeau, 2016). Ae. aegypti from Singapore infected with ZIKV demonstrated high MG infection, resulting in the salivary glands of more than half of the mosquitoes being tested positive for ZIKV (62%) by Day 5, and all mosquitoes potentially infective by Day 10 (Li et al., 2012).
Summary on vector competence
In summary, Ae. aegypti is a most efficient vector for several deadly (e.g. dengue, yellow fever) and debilitating (e.g. chikungunya, Zika) arthropod-borne diseases. The vector competence (VC) for arboviruses is associated with a number of anatomic barriers to productive vector infection. The VC for flaviviruses in Ae. aegypti is thought to be controlled by at least two genes or sets of genes, one controlling the midgut infection barrier (MIB) and the other controlling the midgut escape barrier (MEB). The mosquito susceptibility to DENV, attributable to multiple genetic factors, is usually very high, particularly against DENV-2 as elicited by DS3 and DMEB strains. The ability to overcome the MIB and MEB to transmit DENV-2 JAM1409 is a quantitative trait with multiple genes that likely condition VC and collectively determine the infection rates of mosquito populations. The rate of midgut infection, midgut escape and salivary gland infection generally increases at higher temperature, though may vary amongst different populations. Natural geographic features may also act as a barrier to gene flow in varied Ae. aegypti populations (for VC) for DENV-2.
VC differences among different populations infected with genotypes of DENV result from interactions between mosquito and virus genotypes. At subspecies level, Ae. aegypti aegypti is generally far more efficient in transmitting DENV in urban agglomerations than the sylvatic Ae. aegypti formosus, albeit with a variation in VC.
While Ae. aegypti is unquestionably a much stronger potential transmitter for dengue, yellow fever and Zika viruses, it seems that Ae. albopictus would be a more efficient vector for CHIKV, especially in sylvatic and rural settings. Both mosquitoes are day biters, multiple feeders and capable to transmit several pathogens.
References
Abushouk, A.I., A. Negida and H. Ahmed (2016), “An updated review of Zika virus”, Journal of Clinical Virology, Vol. 84, pp. 53-58.
Ahmad, M., I. Denholm and R.H. Bromilow (2006), “Delayed cuticular penetration and enhanced metabolism of deltamethrin in pyrethroid-resistant strains of Helicoverpa armigera from China and Pakistan”, Pest Management Science, Vol. 62, pp. 805-810.
Aitken, T.H., W.G. Downs and R.E. Shope (1977), “Aedes aegypti strain fitness for yellow fever virus transmission”, The American Journal of Tropical Medicine and Hygiene, Vol. 26, No. 5, pp. 985-989.
Alvarez, L.C. et al. (2015), “Frequency of V1016I and F1534C mutations in the voltage-gated sodium channel gene in Aedes aegypti in Venezuela”, Pest Management Science, Vol. 71, No. 6, pp. 863-869.
Aponte, H.A. et al. (2013), “The pyrethroid resistance status and mechanisms in Aedes aegypti from the Guerrero state, Mexico”, Pesticide Biochemistry and Physiology, Vol. 107, pp. 226-234.
Apostol, B.L., P. Reiter and B.R. Miller (1996), “Population genetics with RAPD-PCR markers: The breeding structure of Aedes aegypti in Puerto Rico”, Heredity, Vol. 76, No. 4, pp. 325-334.
Arensburger, P. et al. (2011), “The mosquito Aedes aegypti has a large genome size and high transposable element load but contains a low proportion of transposon-specific piRNAs”, BMC Genomics, Vol. 12, pp. 606.
Bangs, M.J. (1999), The Susceptibility and Behavior of Anopheles Albimanus Weidemann and Anopheles Vestitipennis Dyar and Knab (Diptera: Culicidae) to Insecticides in Northern Belize, Central America, Uniformed Services University of the Health Sciences, Bethesda (USA).
Bariami, V. et al. (2012), “Gene amplification, ABC transporters and cytochrome P450s: Unraveling the molecular basis of pyrethroid resistance in the dengue vector, Aedes aegypti”, PLoS Neglected Tropical Diseases, Vol. 6, No. 6: e1692.
Belinato, T.A., A.J. Martins and D. Valle (2012), “Fitness evaluation of two Brazilian Aedes aegypti field populations with distinct levels of resistance to the organophosphate temephos”, Memórias do Instituto Oswaldo Cruz., Vol. 107, pp. 916-922.
Bennett, K.E. and B.J. Beaty (2005), “Selection of D2S3, an Aedes aegypti (Diptera: Culicidae) strain with high oral susceptibility to Dengue 2 virus and D2MEB, a strain with a midgut barrier to Dengue 2 escape”, Journal of Medical Entomology, Vol. 42, No. 2, pp. 110-119.
Bennett, K.E. et al. (2002a), “Flavivirus susceptibility in Aedes aegypti”, Archives of Medical Research, Vol. 33, No. 4, pp. 379‑388.
Bennett, K.E. et al. (2002b), “Variation in vector competence for dengue 2 virus among 24 collections of Aedes aegypti from Mexico and the United States”, The American Journal of Tropical Medicine and Hygiene, Vol. 67, No. 1, pp. 85-92.
Biryukova, I. and T. Ye (2015), “Endogenous siRNAs and piRNAs derived from transposable elements and genes in the malaria vector mosquito Anopheles gambiae”, BMC Genomics, Vol. 16, pp. 278.
Black, W.C. 4th et al. (2002), “Flavivirus susceptibility in Aedes aegypti”, Archives of Medical Research, Vol. 33, No. 4, pp. 379-388.
Bosio, C.F. et al. (2005), “Genetic structure of Aedes aegypti populations in Thailand using mitochondrial DNA”, The American Journal of Tropical Medicine and Hygiene, Vol. 72, No. 4, pp. 434-442.
Bosio, C.F. and B.J. Beaty (1998), “Quantitative genetics of vector competence for dengue-2 virus in Aedes aegypti”, The American Journal of Tropical Medicine and Hygiene, Vol. 59, No. 6, pp. 965-970.
Bosio, C.F., R.E. Fulton and M.L. Salasek (2000), “Quantitative trait loci that control vector competence for dengue-2 virus in the mosquito Aedes aegypti”, Genetics, Vol. 156, No. 2, pp. 687-698.
Bracco, J.E. et al. (2007), “Genetic variability of Aedes aegypti in the Americas using a mitochondrial gene: Evidence of multiple introductions”, Memórias do Instituto Oswaldo Cruz, Vol. 102, No. 5, pp. 573-580.
Brogdon, W.G. and J.C. McAllister (1998), “Insecticide resistance and vector control”, Emerging Infectious Diseases, Vol. 4, pp. 605-613.
Brown, S.E. et al. (2001), “Integration of the Aedes aegypti mosquito genetic linkage and physical maps”, Genetics, Vol. 157, No. 3, pp. 1299-1305.
Brown, S.E. et al. (1995), “Toward a physical map of Aedes aegypti”, Insect Molecular Biology, Vol. 4, No. 3, pp. 161‑167.
Campos, J., C.F. Andrade and S.M. Recco-Pimentel (2003), “A technique for preparing polytene chromosomes from Aedes aegypti (Diptera, Culicinae)”, Memórias do Instituto Oswaldo Cruz., Vol. 98, No. 3, pp. 387-390.
Chandre, F. et al. (2000), “Modifications of pyrethroid effects associated with kdr mutation in Anopheles gambiae”, Medical and Veterinary Entomology, Vol. 14, pp. 81-88.
Chareonviriyaphap, T. et al. (1997), “Pesticide avoidance behavior in Anopheles albimanus, a malaria vector in the Americas”, Journal of the American Mosquito Control Association, Vol. 13, pp. 171-183.
Chepkorir, E. et al. (2014), “Vector competence of Aedes aegypti populations from Kilifi and Nairobi for dengue 2 virus and the influence of temperature”, Parasit Vectors, Vol. 7, No. 1, pp. 435.
Christophers, S.R. (1960), Aedes aegypti (L.) The Yellow Fever Mosquito. Its Life History, Bionomics and Structure, Cambridge University Press, Cambridge.
Claudianos, C. et al. (2006), “A deficit of detoxification enzymes: Pesticide sensitivity and environmental response in the honeybee”, Insect Molecular Biology, Vol. 15, pp. 615-663.
Costa-da-Silva, A.L. (2005), “Genetic lineages in the yellow fever mosquito Aedes (Stegomyia) aegypti (Diptera: Culicidae) from Peru”, Memórias do Instituto Oswaldo Cruz, Vol. 100, No. 6, pp. 539-544.
Craig, G.B. Jr and W.A. Hickey (1967), “Current status of the formal genetics of Aedes aegypti”, Bulletin of the World Health Organization, Vol. 36, No. 4, pp. 559-562.
David, J.P. et al. (2006), “Involvement of cytochrome P450 monooxygenases in the response of mosquito larvae to dietary plant xenobiotics”, Insect Biochemistry and Molecular Biology, Vol. 36, pp. 410-420.
Davies, T.G.E. et al. (2007), “DDT, pyrethrins, pyrethroids and insect sodium channels”, IUBMB Life, Vol. 59, pp. 151-162.
Despres, L., J.P. David and C. Gallet (2007), “The evolutionary ecology of insect resistance to plant chemicals”, Trends in Ecolpgy & Evolution, Vol. 22, pp. 298-307.
Diagne, C.T. et al. (2014), “Vector competence of Aedes aegypti and Aedes vittatus (Diptera: Culicidae) from Senegal and Cape Verde archipelago for West African lineages of chikungunya virus”, The American Journal of Tropical Medicine and Hygiene, Vol. 91, No. 3, pp. 635-641.
Diallo, M. et al. (2008), “Vector competence of Aedes aegypti populations from Senegal for sylvatic and epidemic dengue 2 virus isolated in West Africa”, Transactions of the Royal Society of Tropical Medicine and Hygiene, Vol. 102, No. 5, pp. 493-498.
Diallo, M. et al. (2005), “Potential role of sylvatic and domestic African mosquito species in dengue emergence”, The American Journal of Tropical Medicine and Hygiene, Vol. 73, No. 2, pp. 445-449.
Dickson, L.B. et al. (2014), “Vector competence in West African Aedes aegypti Is Flavivirus species and genotype dependent”, PLoS Neglected Tropical Diseases, Vol. 8, No. 10: e3153.
Dueñas, J.C. et al. (2009), “Two different routes of colonization of Aedes aegypti in Argentina from neighboring countries”, Journal of Medical Entomology, Vol. 46, No. 6, pp. 1344-1354.
Esu, E. et al. (2010), “Effectiveness of peridomestic space spraying with insecticide on dengue transmission; Systematic review”, Tropical Medicine and International Health, Vol. 15, pp. 619-631.
Eto, M. (1974), Organophosphorus Pesticides: Organic and Biological Chemistry, CRC Press, Boca Raton.
Failloux, A.B. et al. (2002), “Differentiation of Aedes aegypti populations in French Guiana”, Medical and Veterinary Entomology, Vol. 16, No. 4, pp. 456-460.
Faye, O. et al. (2014), “Molecular evolution of Zika virus during its emergence in the 20th century”, PLoS Neglected Tropical Diseases, Vol. 8: e2636.
Feinson, F.M. and A. Spielman (1980), “Nutrient mediated juvenile hormone secretion in mosquitoes”, Journal of Insect Physiology, Vol. 26, pp. 113-117.
Feyereisen, R (2005), “Insect cytochrome P450”, in L.I. Gilbert, K. Latrou and S.S. Gill (eds.), Comprehensive Molecular Insect Science, Elsevier, Oxford, pp. 1-77.
Figueiredo, R.M. et al. (2008), “Dengue virus type 4, Manaus, Brazil”, Emerging Infectious Diseases, Vol. 14, No. 4, pp. 667-669.
Flores, A.E. et al. (2006), “Mechanisms of insecticide resistance in field populations of Aedes aegypti (L.) from Quintana Roo, Southern Mexico”, Journal of the American Mosquito Control Association, Vol. 22, No. 4, pp. 672-677.
Flores, A.E. et al. (2005), “Elevated alfa-esterases levels associated with permethrin tolerance in Aedes aegypti (L.) from Baja California, Mexico”, Pesticide Biochemistry and Physiology, Vol. 82, pp. 66-78.
Fournier, D. (2005), “Mutations of acetylcholinesterase which confer insecticide resistance in insect populations”, Chemico-Biological Interactions, pp. 157-158 and 257-261.
García-Franco, F. et al. (2002), “Large genetic distances among Aedes aegypti populations along the South Pacific coast of Mexico”, The American Society of Tropical Medicine and Hygiene, Vol. 6, pp. 594‑598.
Gomez-Machorro, C., K.E. Bennett and M.deL. Muñoz (2004), “Quantitative trait loci affecting dengue midgut infection barriers in an advanced intercross line of Aedes aegypti”, Insect Molecular Biology, Vol. 13, No. 6, pp. 637-648.
Gonçalves, C.M. et al. (2014), “Distinct variation in vector competence among nine field populations of Aedes aegypti from a Brazilian dengue-endemic risk city”, Parasites and Vectors, Vol. 7, pp. 320.
Gorrochotegui-Escalante, N. et al. (2005), “Association mapping of segregating sites in the early trypsin gene and susceptibility to dengue-2 virus in the mosquito Aedes aegypti”, Insect Biochemistry and Molecular Biology, Vol. 35, No. 7, pp. 771-788.
Gorrochotegui-Escalante, N. et al. (2002), “Breeding structure of Aedes aegypti populations in Mexico varies by region”, American Journal of Tropical Medicine and Hygiene, Vol. 66, pp. 213–222.
Gorrochotegui-Escalante, N. et al. (2000), “Genetic isolation by distance among Aedes aegypti populations along the northeastern coast of Mexico”, The American Journal of Tropical Medicine and Hygiene, Vol. 62, No. 2, pp. 200‑209.
Grieco, J.P. et al. (2007), “A new classification system for the actions of IRS chemicals traditionally used for malaria control”, PLoS ONE, Vol. 2, No. 8: e716.
Grieco, J.P. et al. (2000), “A comparison study of house entering and exiting behavior of Anopheles vestitipennis (Diptera: Culicidae) using experimental huts sprayed with DDT or deltamethrin in the southern district of Toledo, Belize, C.A.”, Journal of Vector Ecology, Vol. 25, pp. 62–73.
Gubler, D.J. et al. (1979), “Variation in susceptibility to oral infection with dengue viruses among geographic strains of Aedes aegypti”, The American Journal of Tropical Medicine and Hygiene, Vol. 28, No. 6, pp. 1045-1052.
Guillemaud, T. et al. (1997), “Esterase gene amplification in Culex pipiens”, Insect Molecular Biology, Vol. 6, pp. 319‑327.
Guo, X.X. et al. (2013), “Vector competence of Aedes albopictus and Aedes aegypti (Diptera: Culicidae) for DEN2-43 and New Guinea C virus strains of dengue 2 virus”, Acta Tropica, Vol. 128, No. 3, pp. 566‑570.
Harris, A.F., S. Rajatileka and H. Ranson (2010), “Pyrethroid resistance in Aedes aegypti from Grand Cayman”, The American Journal of Tropical Medicine and Hygiene, Vol. 83, pp. 277-284.
Hawkes, N.J. and J. Hemingway (2002), “Analysis of the promoters for the beta-esterase genes associated with insecticide resistance in the mosquito Culex quinquefasciatus”, Biochimica et Biophysica Acta (BBA), Vol. 1574, pp. 51-62.
Hemingway, J. and H. Ranson (2000), “Insecticide resistance in insect vectors of human disease”, Annual Review of Entomology, Vol. 45, pp. 371-391.
Hemingway, J. et al. (2004), “The molecular basis of insecticide resistance in mosquitoes”, Insect Biochemistry and Molecular Biology, Vol. 34, pp. 653-665.
Herrera, F. et al. (2006), “Population genetic structure of the dengue mosquito Aedes aegypti in Venezuela”, Memórias do Instituto Oswaldo Cruz, Vol. 101, No. 6, pp. 625-633.
Hlaing, T. et al. (2009), “Mitochondrial pseudogenes in the nuclear genome of Aedes aegypti mosquitoes: Implications for past and future population genetic studies”, BMC Genetics, Vol. 10, pp. 11.
Horstick, O. et al. (2010), “Dengue vector-control services: how do they work? A systematic literature review and country case studies”, Transactions of the Royal Society of Tropical Medicine and Hygiene, Vol. 104, pp. 379‑386.
Huber, K. et al. (2008), “Aedes aegypti in Senegal: Genetic diversity and genetic structure of domestic and sylvatic populations”, The American Journal of Tropical Medicine and Hygiene, Vol. 79, No. 2, pp. 218-229.
Khambay, B.P.S. (2002), “Pyrethroid insecticides”, Pesticide Outlook, Vol. 13, pp. 49-54.
Kumar, N.P. et al. (2012), “Detection of Chikungunya virus in wild populations of Aedes albopictus in Kerala State, India”, Vector-Borne and Zoonotic Diseases, Vol. 12, No. 10, pp. 907-911.
Lambrechts, L. (2011), “Quantitative genetics of Aedes aegypti vector competence for dengue viruses: Towards a new paradigm?”, Trends in Parasitology, Vol. 27, No. 3, pp. 111-114.
Lambrechts, L. et al. (2011), “Impact of daily temperature fluctuations on dengue virus transmission by Aedes aegypti”, Proceedings of the National Academy of Sciences of the United States of America, Vol. 108, No. 18, pp. 7460-7465.
Lambrechts, L. et al. (2009), “Genetic specificity and potential for local adaptation between dengue viruses and mosquito vectors”, BMC Evolutionary Biology, Vol. 9, pp. 160.
Li, M.I. et al. (2012), “Oral susceptibility of Singapore Aedes (Stegomyia) aegypti (Linnaeus) to Zika virus”, PLOS Neglected Tropical Diseases, Vol. 6, No. 8: e1792.
Lima, E.P. et al. (2011), “Insecticide resistance in Aedes aegypti populations from Ceara, Brazil”, Parasites and Vectors, Vol. 4, pp. 5.
Lima, R.S. Jr and V.M. Scarpassa (2009), “Evidence of two lineages of the dengue vector Aedes aegypti in the Brazilian Amazon, based on mitochondrial DNA ND4 gene sequences”, Genetics and Molecular Biology, Vol. 32, No. 2, pp. 414-422.
Liu, N. et al. (2006), “Pyrethroid resistance in mosquitoes”, Insect Science, Vol. 13, pp. 159-166.
Lozano-Fuentes, S. et al. (2012), “The dengue virus mosquito vector Aedes aegypti at high elevation in Mexico”, The American Journal of Tropical Medicine and Hygiene, Vol. 87, No. 5, pp. 902-909.
Lozano-Fuentes, S. et al. (2009), “The neovolcanic axis is a barrier to gene flow among Aedes aegypti populations in Mexico that differ in vector competence for Dengue 2 virus”, PLoS Neglected Tropical Diseases, Vol. 3, No. 6: e468.
Lumjuan, N. et al. (2007), “The Aedes aegypti glutathione transferase family”, Insect Biochemistry and Molecular Biology, Vol. 37, pp. 1026-1035.
Madhu, S.K. and V.A. Vijayan (2009), “Laboratory evaluation of a juvenile hormone mimic, pyriproxyfen on Culex quinquefasciatus Say and Aedes aegypti Linn. at Mysore, India”, The Journal of Communicable Diseases, Vol. 41, No. 3, pp. 169-174.
Maestre-Serrano, R. et al. (2014), “Susceptibility to insecticides and resistance mechanisms in Aedes aegypti from the Colombian Caribbean Region”, Pesticide Biochemistry and Physiology, No. 116, pp. 63‑73.
Malaithong, N. et al. (2010), “Human landing patterns of Anopheles dirus sensu lato (Diptera: Culicidae) in experimental huts treated with DDT or deltamethrin”, Journal of Medical Entomology, Vol. 47, pp. 823-832.
Marcombe, S. et al. (2013), “Insecticide-driven patterns of genetic variation in the dengue vector Aedes aegypti in Martinique Island”, PLoS One, Vol. 8: e77857.
Marcombe, S. et al. (2012), “Insecticide resistance in the dengue vector Aedes aegypti from Martinique: Distribution, mechanisms and relations with environmental factors”, PLoS One, Vol. 7: e30989.
Marcombe, S. et al. (2009), “Exploring the molecular basis of insecticide resistance in the dengue vector Aedes aegypti: A case study in Martinique Island (French West Indies)”, BMC Genomics, Vol. 10, pp. 494.
Martins, A.J. et al. (2009a), “Frequency of Val1016Ile mutation in the voltage-gated sodium channel gene of Aedes aegypti Brazilian populations”, Tropical Medicine & International Health, Vol. 14, pp. 1351-1355.
Martins, A.J. et al. (2009b), “Voltage-gated sodium channel polymorphism and metabolic resistance in pyrethroid-resistant Aedes aegypti from Brazil”, The American Journal of Tropical Medicine and Hygiene, Vol. 81, pp. 108-115.
McAllister, J.C., M.S. Godsey and M.L. Scott (2012), “Pyrethroid resistance in Aedes aegypti and Aedes albopictus from Port-au-Prince, Haiti”, Journal of Vector Ecology, Vol. 37, pp. 325-332.
McClelland, G.A.H. (1962), “Sex-linkage in Aedes aegypti”, Transaction of the Royal Society of Tropical Medicine and Hygine, Vol. 56, No. 4.
McDonald, P.T. and K.S. Rai (1970), “Correlation of linkage groups with chromosomes in the mosquito, Aedes aegypti”, Genetics, Vol. 66, pp. 475–485.
Mercado-Curiel, R.F., W.C. Black 4th and M.deL. Muñoz (2008), “A dengue receptor as possible genetic marker of vector competence in Aedes aegypti”, BMC Microbiology, Vol. 8, pp. 118.
Miller, B.R. and C.J. Mitchell (1991), “Genetic selection of a flavivirus-refractory strain of the yellow fever mosquito Aedes aegypti”, The American Journal of Tropical Medicine and Hygiene, Vol. 45, No. 4, pp. 399‑407.
Moncayo, A.C. et al. (2004), “Dengue emergence and adaptation to peridomestic mosquitoes”, Emerging Infectious Diseases, Vol. 10, No. 10, pp. 1790-1796.
Moore, M. et al. (2013), “Dual African origins of global Aedes aegypti s.l. populations revealed by mitochondrial DNA”, PLoS Neglected Tropical Diseases, Vol. 7, No. 4: e2175.
Mousson, L. et al. (2005), “Phylogeography of Aedes (Stegomyia) aegypti (L.) and Aedes (Stegomyia) albopictus (Skuse) (Diptera: Culicidae) based on mitochondrial DNA variations”, Genetic Research , Vol. 86, pp. 1–11.
Muñoz, M.deL. et al. (2013a), “Gene flow pattern among Aedes aegypti populations in Mexico”, Journal of the American Mosquito Control Association, Vol. 29, No. 1, pp. 1-18.
Muñoz, M.deL. et al. (2013b), “Proteomic identification of dengue virus binding proteins in Aedes aegypti mosquitoes and Aedes albopictus cells”, Biomed Res Int, Article ID: 875958.
Munstermann, L.E. and G.B. Craig Jr. (1979), “Genetics of Aedes aegypti: Updating the linkage map”, Journal of Heredity, Vol. 70, pp. 291‑296.
Nauen, R. (2006), “Insecticide mode of action: Return of the ryanodine receptor”, Pest Management Science, Vol. 62, pp. 690-692.
Nauen, R. and T. Bretschneider (2002), “New modes of action of insecticides”, Pesticide Outlook, Vol. 13, pp. 241‑245.
Navarro, J.C. et al. (2010), “Highest mosquito records (Diptera: Culicidae) in Venezuela”, Revista de Biología Tropical, Vol. 58, No. 1, pp. 245‑1254.
Nene, V. et al. (2007), “Genome sequence of Aedes aegypti, a major arbovirus vector”, Science, Vol. 316, No. 5832, pp. 1718‑1723.
Oakeshott, J.G. et al. (2005), “Biochemical genetics and genomics of insect esterases”, in L.I. Gilbert, K. Latrou and S.S. Gill (eds.), Comprehensive Molecular Insect Science Pharmacology, Vol. 5, pp. 309‑381.
Paduan, K.S. and P.E. Ribolla (2008), “Mitochondrial DNA polymorphism and heteroplasmy in populations of Aedes aegypti in Brazil”, Journal of Medical Entomology, Vol. 45, No. 1, pp. 59-67.
PAHO (1994), Dengue and Dengue Hemorrhagic Fever in the Americas: Guidelines for Prevention and Control, PAHO Scientific Publication 548, Pan American Health Organization, Washington DC.
Paupy, C. et al. (2012), “Genetic structure and phylogeography of Aedes aegypti, the dengue and yellow-fever mosquito vector in Bolivia”, Infection, Genetics and Evolution, Vol. 12, No. 6, pp. 1260-1269.
Paupy, C. et al. (2008), “Gene flow between domestic and sylvan populations of Aedes aegypti (Diptera: Culicidae) in North Cameroon”, Journal of Medical Entomology, Vol. 45, No. 3, pp. 391-400.
Paupy, C. et al. (2004), “Comparisons of amplified fragment length polymorphism (AFLP), microsatellite, and isoenzyme markers: Population genetics of Aedes aegypti (Diptera: Culicidae) from Phnom Penh (Cambodia)”, Journal of Medical Entomology, Vol. 41, No. 4, pp. 664-671.
Polsomboon, S. et al. (2008), “Biting patterns of Anopheles minimus complex (Diptera: Culicidae) in experimental huts treated with DDT and deltamethrin”, Journal of Vector Ecology, Vol. 33, pp. 285-292.
Ponce-Garcia, G. et al. (2009), “Recent rapid rise of a permethrin knock down resistance allele in Aedes aegypti in Mexico”, PLoS Neglected Tropical Diseases, Vol. 3: e531.
Powell, J.R. and W.J. Tabachnick (2013), “History of domestication and spread of Aedes aegypti – A review”, Memórias do Instituto Oswaldo Cruz, Vol. 108, pp. 11-17.
Puinean, A.M. et al. (2010), “Amplification of a cytochrome P450 gene is associated with resistance to neonicotinoid insecticides in the aphid Myzus persicae”, PLoS Genet, Vol. 6: e1000999.
Rai, K.S. (1963), “A comparative study of mosquito karyotypes”, Annals of the Entomological Society of America, Vol. 56, pp. 160‑170.
Ranson, H. and J. Hemingway (2005), “Mosquito glutathione transferases”, Methods in Enzymology, Vol. 401, pp. 226-241.
Ranson, H. et al. (2002), “Evolution of supergene families associated with insecticide resistance”, Science, Vol. 298, pp. 179-181.
Reiter, P. and D.J. Gubler (1997), “Surveillance and control of urban dengue vectors”, in D.J. Gubler and G. Kuno (eds.), Dengue and Dengue Hemorrhagic Fever, CABI Publishing, Cambridge, pp. 425-462.
Ribeiro, J.M. et al. (2007), “An annotated catalogue of salivary gland transcripts in the adult female mosquito, Aedes aegypti”, BMC Genomics, Vol. 8, No. 6.
Richard, V., T. Paoaafaite and V-M. Cao-Lormeau (2016), “Vector competence of French Polynesian Aedes aegypti and Aedes polynesiensis for Zika virus”, PLoS Neglected Tropical Diseases, Vol. 10, No. 9: e0005024.
Rinkevich, F.D., Y.Z. Du and K. Dong (2013), “Diversity and convergence of sodium channel mutations involved in resistance to pyrethroids”, Pesticide Biochemistry and Physiology, Vol. 106, pp. 93-100.
Roberts, D.R. and W.D. Alecrim (1991), “Behavioral response of Anopheles darlingi to DDT-sprayed house walls in Amazonia”, Bulletin of the Pan American Health Organization, Vol. 25, pp. 210-217.
Rosen, L. et al. (1985), “Comparative susceptibility of mosquito species and strains to oral and parenteral infection with dengue and Japanese encephalitis viruses”, The American Journal of Tropical Medicine and Hygiene, Vol. 34, No. 3, pp. 603‑615.
Rozendaal, J.A. et al. (1989), “Behavioral responses of Anopheles darlingi in Suriname to DDT residues on house walls”, Journal of the American Mosquito Control Association, Vol. 5, pp. 339-350.
Saavedra-Rodriguez, K. et al. (2007), “A mutation in the voltage-gated sodium channel gene associated with pyrethroid resistance in Latin American Aedes aegypti”, Insect Molecular Biology, Vol. 16, No. 6, pp. 785‑798.
Saito, K. and M.C. Siomi (2010), “Small RNA-mediated quiescence of transposable elements in animals”, Developmental Cell, Vol. 19, No. 5, pp. 687-697.
Senti, K.A. and J. Brennecke (2010), “The piRNA pathway: A fly's perspective on the guardian of the genome”, Trends in Genetics, Vol. 26, No. 12, pp. 499-509.
Service, M. (2012), Medical Entomology for Students, 5th Ed., Cambridge University Press, New York, pp. 303.
Severson, D.W. and S.K. Behura (2012), “Mosquito genomics: Progress and challenges”, Annual Review of Entomology, Vol. 57, pp. 143-166.
Severson, D.W. et al. (2004), “Comparative genome analysis of the yellow fever mosquito Aedes aegypti with Drosophila melanogaster and the malaria vector mosquito Anopheles gambiae”, Journal of Heredity, Vol. 95, No. 2, pp. 103-113.
Severson, D.W. et al. (2002), “Linkage map organization of expressed sequence tags and sequence tagged sites in the mosquito, Aedes aegypti”, Insect Molecular Biology., Vol. 11, No. 4, pp. 371-378.
Severson, D.W. et al. (1995), “Restriction fragment length polymorphism mapping of quantitative trait loci for malaria parasite susceptibility in the mosquito Aedes aegypti”, Genetics, Vol. 139, No. 4, pp. 1711-1717S.
Severson, D.W. et al. (1994), “Chromosomal mapping of two loci affecting filarial worm susceptibility in Aedes aegypti”, Insect Molecular Biology, Vol. 3, No. 2, pp. 67-72.
Severson, D.W. et al. (1993), “Linkage map for Aedes aegypti using restriction fragment length polymorphisms,” Journal of Heredity, Vol. 84, No. 4, pp. 241-247.
Sharakhova, M.V. et al. (2011), “Arm-specific dynamics of chromosome evolution in malaria mosquitoes”, BMC Evolutionary Biology, Vol. 11, pp. 91.
Sharma, G.P. et al. (1978), “A preliminary map of the salivary gland chromosomes of Aedes (Stegomyia) aegypti (Culicidae, Diptera)”, Cytobios, Vol. 22, pp. 169-178.
Sieglaff, D.H., K.A. Duncan and M.R. Brown (2005), “Expression of genes encoding proteins involved in ecdysteroidogenesis in the female mosquito, Aedes aegypti”, Insect Biochemistry and Molecular Biology, Vol. 35, pp. 471-490.
Siller, Q. et al. (2011), “Update on the frequency of Ile1016 mutation in voltage-gated sodium channel gene of Aedes aegypti in Mexico”, Journal of the American Mosquito Control Association, Vol. 27, pp. 357-362.
Smith, A. (1965), “A verandah-trap hut for studying the house-frequenting habits of mosquitos and for assessing insecticides. 2. The effect of dichlorvos (DDVP) on egress and mortality of Anopheles gambiae Giles and Mansonia uniformis (Theo.) entering naturally”, Bulletin of Entomological Research, Vol. 56, pp. 275-282.
Soderlund, D.M. and D.C. Knipple (2003), “The molecular biology of knockdown resistance to pyrethroid insecticides”, Insect Biochemistry and Molecular Biology, Vol. 33, pp. 563-577.
Sylla, M. et al. (2009), “Gene flow, subspecies composition, and dengue virus-2 susceptibility among Aedes aegypti collections in Senegal”, PLoS Neglected Tropical Diseases, Vol. 3, No. 4: e408.
Tabachnick, W.J. and J.R. Powell (1979), “A world-wide survey of genetic-variation in the yellow-fever mosquito, Aedes aegypti”, Genetical Research, Vol. 34, No. 3, pp. 215-229.
Tabachnick, W.J. et al. (1985), “Oral infection of Aedes aegypti with yellow-fever virus – Geographic variation and genetic considerations”, American Journal of Tropical Medicine and Hygiene, Vol. 34, No. 6, pp. 1219-1224.
Tardieux, I. et al. (1990), “Variation among strains of Aedes aegypti in susceptibility to oral infection with dengue virus type 2”, The American Journal of Tropical Medicine and Hygiene, Vol. 43, No. 3, pp. 308-313.
Timoshevskiy, V.A. et al. (2014), “Genomic composition and evolution of Aedes aegypti chromosomes revealed by the analysis of physically mapped supercontigs”, BMC Biology, Vol. 12, pp. 27.
Timoshevskiy, V.A. et al. (2013), “An integrated linkage, chromosome, and genome map for the yellow fever mosquito Aedes aegypti”, PLoS Neglected Tropical Diseases, Vol. 7, No. 2, pp. 2052.
Urdaneta-Marquez, L. et al. (2008), “Genetic relationships among Aedes aegypti collections in Venezuela as determined by mitochondrial DNA variation and nuclear single nucleotide polymorphisms”, The American Journal of Tropical Medicine and Hygiene, Vol. 78, No. 3, pp. 479‑491.
Valles, S.M., K. Dong and R.J. Brenner (2000), “Mechanisms responsible for cypermethrin resistance in a strain of German cockroach, Blattella germanica”, Pesticide Biochemistry and Physiology, Vol. 66, pp. 195-205.
Vazeille-Falcoz, M. et al. (1999), “Variation in oral susceptibility to dengue type 2 virus of populations of Aedes aegypti from the islands of Tahiti and Moorea, French Polynesia”, The American Journal of Tropical Medicine and Hygiene, Vol. 60, No. 2, pp. 292-299.
Vega-Rúa, A. et al. (2014), “High level of vector competence of Aedes aegypti and Aedes albopictus from ten American countries as a crucial factor in the spread of Chikungunya virus”, Journal of Virology, Vol. 88, No. 11, pp. 6294-6306.
Vontas, J. et al. (2012), “Insecticide resistance in the major dengue vectors Aedes albopictus and Aedes aegypti”, Pesticide Biochemistry and Physiology, Vol. 104, pp. 126-131.
Watts, D.M. et al. (1987), “Effect of temperature on the vector efficiency of Aedes aegypti for dengue 2 virus”, The American Journal of Tropical Medicine and Hygiene, Vol. 36, No. 1, pp. 143-152.
Weill, M. et al. (2003), “Comparative genomics: Insecticide resistance in mosquito vectors”, Nature, Vol. 423, pp. 136-137.
WHO (2012a), WHO Recommended Insecticides for Space Spraying Against Mosquitoes, World Health Organization, Geneva, www.who.int/whopes/Insecticides_for_space_spraying_Jul_2012.pdf.
WHO (2012b), Global Plan for Insecticide Resistance Management in Malaria Vectors (GPIRM), World Health Organization, Geneva.
WHO (2009), WHO Recommended Insecticides for Indoor Residual Spraying Against Malaria Vectors, World Health Organization, Geneva, www.who.int/whopes/Insecticides_IRS_Malaria_09.pdf.
WHOPES (2005), Guidelines for Laboratory and Field Testing of Mosquito Larvicides, World Health Organization Pesticide Evaluation Scheme, Geneva.
Zhong, D. et al. (2006), “Amplified fragment length polymorphism mapping of quantitative trait loci for malaria parasite susceptibility in the yellow fever mosquito Aedes aegypti”, Genetics, Vol. 173, No. 3, pp. 1337-1345.