This chapter provides background on the transformation of freight transport and scopes the impacts of potential disruptions such as e-commerce, 3D printing, new international trade routes, autonomous trucks and high capacity vehicles. Also explored are scenarios that combine different disruptions, quantifying the impacts of more technology-oriented changes, logistic or exogenous transformation and all disruptions combined with high policy ambitions. The first section revisits the current ambition and high ambition scenarios for a more in-depth look at their freight-related features.
ITF Transport Outlook 2019
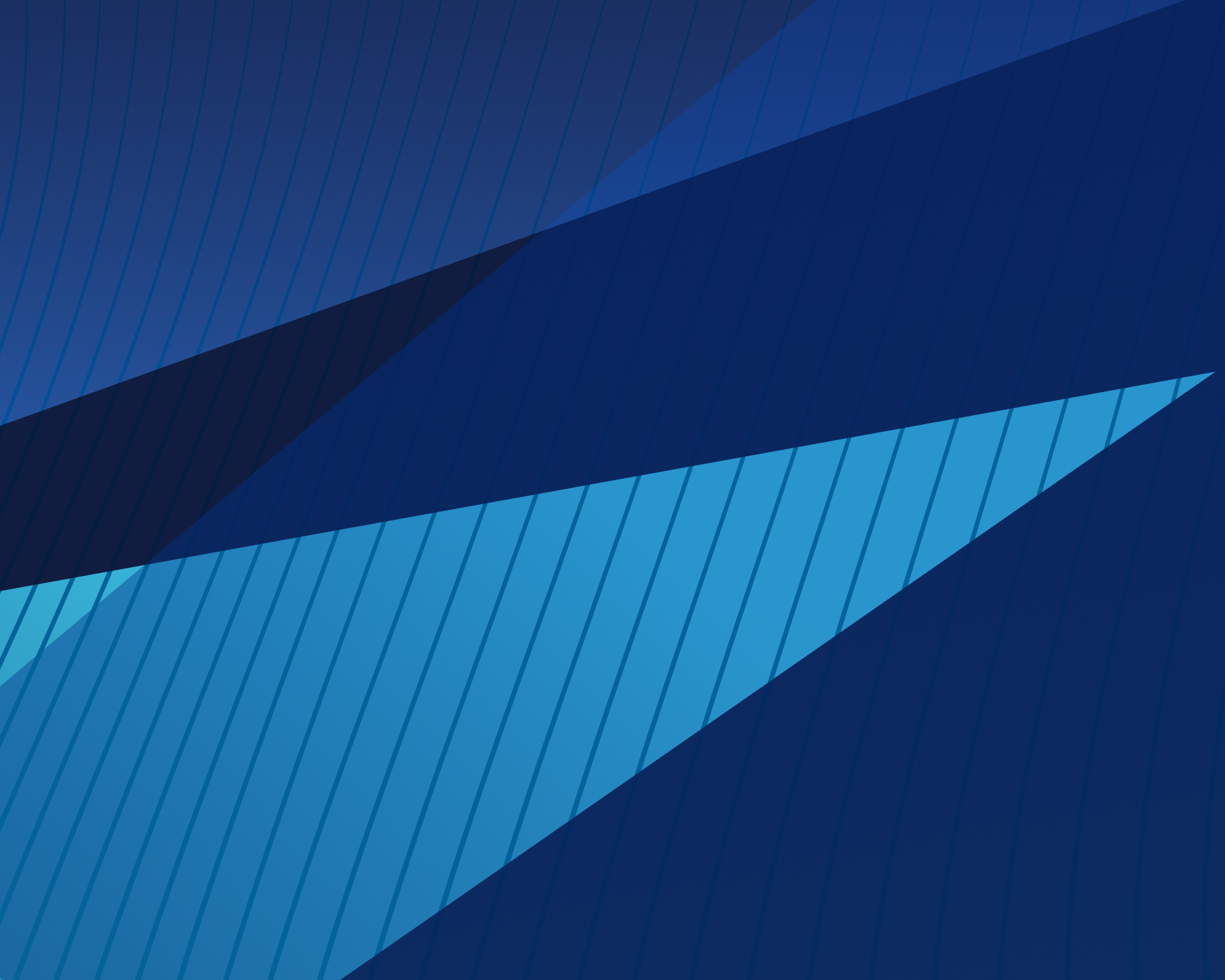
Chapter 5. Disruptions in freight transport
Abstract
Strong growth expected in freight transport amid high uncertainty
Freight volumes will continue to grow strongly, with global freight demand projected to triple between 2015 and 2050. At the same time, freight transport and logistics are undergoing major transformations, and these will likely be even more disruptive in the future. Technology, business models, consumer behaviour, shifts in trade patterns and other factors all contribute to a changing transport landscape and how they play out can have a substantial impact on the projected growth.
Although unlikely, new opportunities for commercial shipping could open in light of the decrease of the extent of ice cover in the Artic sea, which would shorten distances considerably from Asia to both Europe and North America (ITF, 2018[1]). Large transcontinental infrastructure projects may establish alternative routes between major trade partners in East Asia and Europe, while also increasing access to markets in Central Asia and other regions including Africa. This can have an impact on port activity and the way surface transport infrastructure is used. Some parts of current road, rail and river networks could experience major reductions in traffic while others would see sharp increases.
E-commerce has been steadily growing and is predicted to increase further. Greater ease of purchase and returns can increase demand and foster a trend towards more individualised, small-scale deliveries, leading to more freight transport and increasing the share of relatively carbon-intensive modes, such as air and road.
Other disruptions can come from increased vehicle automation. The ability to decrease or totally remove labour costs and use vehicles in more flexible ways can significantly cut transport costs and revolutionise the freight transport market, not least by pushing up demand for road freight and shifting freight from rail and inland waterways onto roads.
Great uncertainty surrounds the extent to which re-shoring and 3D printing will dampen future growth of freight transport. If adopted extensively, both can affect the type of goods moved, decrease the distances between production and consumption centres and thus fundamentally alter today’s long and complex supply chains. A significant decrease in the total value of internationally traded goods can greatly reduce sea and air transport volumes.
High capacity vehicles (HCV) that carry bigger loads than regular trucks are already in operation in some OECD countries, for instance in Finland and Australia. They could contribute to lowering emissions, limiting congestion and reducing overall transportation costs while increasing safety. But there are also caveats: Like other cost saving measures, HCVs could cause a rebound effect: If they cause a reverse modal shift from rail to road transport, the net impact on emissions will be negative above a certain threshold.
Zero or near zero-emissions propulsion for long-haul heavy freight trucks will not come into widespread use in the short to medium term. However, such solutions would need to be in general use by 2050 or earlier to reach the internationally agreed climate change targets. Decarbonising technologies for heavy-duty long-haulage currently foreseeable are the direct supply of electric energy to the vehicle (“electric roads”), hydrogen and possibly electric batteries. Their widespread adoption by 2050 could lead to a decrease of total freight related emissions, although this would also require zero-carbon generation of electric power and hydrogen.
Table 5.1. Current and high ambition scenario specifications for freight transport
Mitigation measures |
|||
---|---|---|---|
Assumption |
Current ambition scenario |
High ambition scenario |
|
|
International trade, coal and oil consumption |
Moderate reductions following the OECD ENV-Linkages model |
Accelerated reductions. Coal and oil trade volumes decrease by 50% and 33%, respectively, by 2035 |
|
Logistics efficiency |
Moderate efficiency improvements following the IEA new policies scenario |
High efficiency improvements following the IEA EV30@30 scenario |
|
Efficiency improvements and electric vehicles |
Moderate efficiency improvements and electric vehicle uptake following the IEA new policies scenario |
High efficiency improvements and electric vehicle uptake following the IEA EV30@30 scenario |
Potentially disruptive developments |
|||
Assumption |
Current ambition scenario |
High ambition scenario |
|
|
E-commerce |
Slight increase in urban freight demand (5% in more developed regions by 2050) |
|
|
3D printing |
No change from current uptake |
|
|
New trade routes |
Planned infrastructure capacity and connectivity improvements occur |
|
|
Energy transition for long-distance heavy freight |
Following IEA new policies scenario |
Energy transition for long-distance heavy freight |
|
High capacity vehicles |
5% increase in the uptake of high capacity vehicles for inter-urban road freight. HCV allow a 50% increase in truck loads and lower costs by 20% per tonne-kilometre |
|
Additional underlying assumptions |
|||
GDP |
Following ECO-OECD forecasts |
||
Population |
Following UN World Population Prospects |
||
Transport network (Sea, Road, Rail, Inland waterways, Air) |
Existing networks (2015). Planned ports expansion (increase in capacity) and some new road and rail links in Central Asia. |
||
Transport Costs |
Current generalised costs per mode, calibrated per country |
||
Border-Crossing |
Current assessment. Planned improvements in Central Asia. |
In order to estimate the impacts of each of these potential disruptions, individually as well as in combination, simulations for this Transport Outlook were carried out using an upgraded version of the ITF freight model that now integrates the (previously distinct) surface and international freight models. This brings greater consistency to the estimates produced, extends the ability to evaluate shifts in mode choice and allocates all freight volumes – domestic and international - to a multi-modal, routable and global network that covers sea, road, rail, air and inland waterways.
The network contains 7 707 “centroids”, where consumption and production of goods takes place. Of these, 404 represent the origin and destination for international trade flows and 7 303 domestic flows. The 253 499 links contain information on capacity, travel time, distance, costs per tonne-kilometre (t-km) and border crossing times. The updated ITF freight model is also used to estimate the impact of policies under the current ambition and high ambition scenarios presented in Chapter 2. Table 5.1 summarises the assumptions for both scenarios regarding freight transport.
Elasticity of global trade to GDP has decreased since the 2008 financial crisis (WTO, 2018[4]). This has coincided with a rising number of trade disputes and increased protectionism (OECD, WTO and UNCTAD, 2018[5]). Should these persist, the impact could transform global supply chains and affect the volume of goods, the types of commodities, mode choice and distances travelled. This potential disruption should not be overlooked. Although it is not directly considered in this report, the simulation of the effects of 3D printing can provide some insights, as it is generally associated with re-shoring.
Disruptions represent qualitative transformations which can lead to paradigm shifts in manufacturing, transport, logistics and even land use. Their exact consequences are inherently uncertain, however. In this Transport Outlook, the potential impacts of these disruptions are quantified within a coherent and consistent modelling framework, reflecting the current knowledge on these topics and exploring the upper limits of these potential disruptions. Yet the results presented here are based on assumptions of future developments for which there is no historical precedent, and thus provide only a range for the potentially disruptive impacts on transport.
The mitigation potential of known freight transport policies and measures
Global freight transport accounts for 36% of the total transport CO2 emissions today. Projections see its share of transport’s carbon footprint increase to 48% by 2050 under the current ambition scenario. The figures show not only freight’s current sizeable contribution towards CO2 emissions, but underlines its increasing relevance towards the overall decarbonisation effort and the need to move decarbonising freight transport up on policy agendas.
The insufficient advances in reducing CO2 emissions from freight transport are partly due to technical reasons that make it hard to decarbonise the sector. It will thus not be possible to decrease emissions from freight without added policy attention that support the deployment of both short-term measures that are relatively easy to adopt and more ambitious initiatives like wide-spread introduction of alternative fuels (ITF, 2018[6]; ITF, 2018[1]).
Even the deployment of ambitious policies will not suffice to reduce global CO2 emissions below their 2015 level by the year 2050. Ambitious policy targets need to be associated with the deployment of a full array of logistical and technological measures combined with exogenous changes that may curb the rate of demand growth.
Important differences exist across regions and sectors. High ambition policies can decrease emissions for surface modes in Europe by 50% and by 41% in OECD countries to 2050. But in Asia and Africa CO2 emissions will continue to increase, largely due to higher growth of transport activity and lower technological and logistical efficiency in several countries in these regions. Given the different geographic, economic, regulatory and infrastructure conditions, a set of regionally targeted freight decarbonisation strategies will be more suitable than a universal approach.
Carbon emissions from air and sea transport continue to grow to 2050 with currently implemented policies. This is because growing international trade increases demand for these modes more strongly. A second factor is that considerable progress in technical efficiency and carbon intensity reductions would be needed. Surface transport is easier to regulate by national governments or inter-regional associations of governments, whereas air and sea transport operate at a more transnational, even transcontinental, level. Although the International Maritime Organisation has announced absolute emission reduction targets, these can only be realised if far-going measures will be implemented, e.g. related to ship speed, energy efficiency and alternative fuels. Such a decarbonisation scenario is suggested in ITF (2018[1]).
If decarbonising efforts indeed increase their pace, in transport and sectors such as energy production decarbonise, the volumes of fossil fuels moved will also decrease sharply. The underlying international trade estimates in the current ambition scenario already include a decrease of the relative importance of fossil fuels compared to other types of commodities. The high ambition scenario assumes a more drastic decrease in volumes of coal and oil transported by 2035 (see Table 5.1).
A major difference between the current and high ambition scenarios are the respective assumptions about the efficiency of logistics and vehicle technology (e.g. see Figure 5.1 and Figure 5.2). The current ambition scenario assumes targets and policies announced by governments up to date following the IEA’s new policies scenarios (NPS). The high ambition scenario, in contrast, assumes that 30% of new vehicles sold by 2030 are electric and that an extensive electrification of railways takes place.
Scaling up decarbonisation measures for road freight transport that have already been tested and are comparatively easy to introduce is one of the most immediate actions required. For urban freight operations, alternative fuels already provide a viable commercial solution or shortly will. In both scenarios, more so on the high ambition, significant decreases in the carbon emissions for urban operations are assumed. Policy can foster measures such as the adoption of alternative fuels for urban logistics operations through pricing mechanisms and other incentives, stricter emission standards, zero emissions zones, recharging infrastructure and policies geared towards adoption of alternative fuels by large fleets.
Improving logistics practices also plays an important role in freight decarbonisation, with a potential to reduce emissions by as much as 30‑50% (ITF, 2018[6]). However, there are only few case studies that substantiate costs and benefits. Anecdotal evidence exists of collaborative logistics schemes, but so far such practices have not been adopted at scale. Available data does not show a significant contribution of logistic solutions to CO2 emissions reductions. Moreover, there is little data to properly assess the current situation and estimate the impacts that logistic solutions might have.
The difficulty to increase logistical efficiency in an urban environment is reflected in the actual decrease in average loads assumed in the current ambition scenario. This results in higher levels of congestion which, unlike emissions, cannot be offset by increased use of alternative fuels. Potential gains in logistic efficiency are set out in box 5.1.
Figure 5.1. Carbon intensity of urban and non-urban trucks in the current and high ambition scenarios
Box 5.1. Optimised logistics for low-carbon freight transport
Logistic solutions have significant decarbonisation potential, even though more attention is paid to technological solutions for reducing CO2 emissions from road freight transport (McKinnon, 2018[7]). Overall, more efficient freight operations could reduce CO2 emissions from freight transport by between 45% and 67% (Holguín-Veras et al., 2016[8]). Logistical decarbonisation measures maximise the amount of freight tonnes transported per kilometre driven. They can include optimised routes, relaxed delivery windows, and shared assets between firms (ITF, 2018[6]).
Route optimisation alone could generate energy savings on the order of 1‑5% (IEA, 2017[9]). Extending delivery windows can mitigate emissions by reducing vehicle speeds, consolidating trips and improving payload capacity use (McKinnon, 2016[10]). Energy savings from re-timing urban deliveries are likely to be around 5‑10% (IEA, 2017[9]), although they are difficult to estimate. Re-timing deliveries can also help to reduce congestion, save time, reduce stress for staff, improve safety and enhance reliability.
A number of studies have found that narrow delivery windows can hinder efforts to increase capacity use (Route Monkey and WBCSD, 2016[11]; Transport & Mobility Leuven, 2017[12]; Sánchez-Díaz, Georén and Brolinson, 2017[13]). Re-timing deliveries goes against current market trends of increasingly on-demand shipping options, however. The cost savings involved in off-peak deliveries are attractive for freight operators, yet customers will require incentives to accept relaxed delivery windows. Local restrictions on off-hour deliveries in residential areas, often motivated by noise concerns, pose another obstacle to more flexible delivery windows. Incentives for freight operators to adopt low-noise technologies and vehicles could address this.
Supply chain collaboration can further decrease the energy consumption, costs and emissions of freight operations. It involves freight operators sharing vehicles, warehouses or workers to increase efficiency of deliveries. Digital tools can facilitate co-operation in an atomised sector. The impacts of collaboration and asset-sharing are arguably substantial, though difficult to quantify. Antitrust laws can hinder moves towards horizontal collaboration in logistics. Digital collaboration platforms, operated by neutral trusted third parties, offer a promising pathway to overcome these hurdles.
The physical internet could offer an operational revolution in the long run. The term stands for an open global logistics system in which asset-sharing and collaboration is coupled with modular standardised packaging units (Montreuil, 2011[14]). In the physical internet, standardised exchange protocols (e.g. with respect to parcel size and accompanying data) allow goods to be transported across modes on a common network in much the same way that information circulates on the digital internet, enabling large efficiency gains.
Challenges to implementing logistical decarbonisation measures include a lack of available data and research available regarding freight movements and their impacts, and the alignment of decarbonisation measure outcomes with the aims of profit-seeking firms and the broader market priorities related to maintaining and improving consumer services.
Figure 5.2. Average loads for urban and non-urban trucks in the current and high ambition scenarios
Box 5.2. Towards Road Freight Decarbonisation
The freight sector is an important factor for economic growth. Road freight is a flexible way to deliver goods, able to access most regions and is cost competitive with other modes. It will remain an irreplaceable transport mode, particularly for last-mile delivery. Road transport currently represents 18% of total freight activity and 57% of CO2 emissions related to freight. Its share of transport sector emissions is projected to grow from 20% to 24% by 2050, barring disruptive innovation.
Within the framework of the Decarbonising Transport initiative led by the ITF, a workshop and an expert survey were conducted with the aim of identifying policies which are both cost-effective in mitigating the carbon footprint of road freight and improve the sector’s operational efficiency. The main recommendations are summarized below:
Broaden access to relevant data and improve their analytical uses for policies to decarbonise road freight transport
Scale up tested and low-barrier decarbonisation measures for road freight transport
Seek ways to overcome regulatory barriers to collaboration in the logistics sector
Demonstrate the business case for investing in decarbonisation measures
In the mid to long-term, mainstream the use of alternative fuels with ultra-low or zero CO2 emissions for road freight transport
Tailor decarbonising pathways to the economic and geographical realities of different country groups
Further insights can be found in ITF (2018[6])
Trucks that carry higher loads increase the efficiency of road freight operations. This does not always require high capacity vehicles (HCV). Renewing the fleet of trucks in developing countries with newer and larger vehicles (e.g. the standard heavy truck sizes in use in Europe or the United States) per se would have a significant impact.
The combined effect of improved technology and enhanced logistic efficiency, which is partly counteracted by a move towards lighter commodities with lower average loads, leads to a significant reduction of carbon intensity across all modes on the high ambition scenario. Surface modes in general and rail in particular achieve higher reductions than air and sea (see Table 5.2). In this chapter the disruptions in aviation technology presented in Chapter 4 for non-urban passenger transport were not considered. Though there are still efficiency gains.
Modal shift from road to less carbon intensive modes such as rail is another long-debated option to decarbonise freight. Indeed, road freight accounts for 18% of total freight volumes, but has the highest share of emissions emitting more than half of freight transport emissions. Rail transport, on the other hand, is already widely electrified in regions such as Europe or Japan and further electrification of railway lines is a relatively straightforward option to decarbonise freight transport.
This said, road freight transport offers a level of flexibility, accessibility and overall service level at competitive costs that make a pure modal shift difficult. In Europe, modal shift to rail has remained far below expectations, and there are several structural reasons for this (Crozet and Woodburn, 2014[15]). The European Union target, was to shift 30% of road freight over 300 km distance to rail and inland waterways by 2030, and 50% by 2050 (European Commission, 2011[16]).
Achieving the 2030 target would mean that the overall rail share would be close to 40% and road just above 50% according to Tavasszy and Meijeren (2011[17]). Yet it will be a challenge in itself for rail to keep its current mode share, given that demand for some core commodities currently moved by rail (i.e. heavy bulk materials such as coal and other fossil fuels) will probably decrease and more fuel-efficient trucks could narrow the gap in carbon intensity between long-haul road and rail freight. Though as seen in Table 5.2 in the high ambition scenario, presumably rail will decrease its already low carbon intensity more than any other mode.
In other regions, modal shift might be an option with greater potential than Europe. Rail becomes more attractive where longer distances need to be covered, the coastline is shorter, fewer ports are available, and transport corridors are more concentrated (e.g. in India, China or South Africa).
Table 5.2. Projected fall in freight transport carbon intensity, 2015 to 2030/2050
High ambition scenario, percentage decrease in tonnes of CO2 emitted per tonne-kilometre
Year |
Sea |
Air |
Non-urban road |
Urban road |
Rail |
Inland waterways |
---|---|---|---|---|---|---|
2015‑2030 |
-23 |
-29 |
-29 |
-27 |
-39 |
-37 |
2015‑2030 |
-56 |
-51 |
-63 |
-76 |
-80 |
-68 |
Figure 5.3. Projected surface freight CO2 emissions by region and scenario, 2030-50
Implementing highly ambitious policies can significantly decrease emissions. Total freight transport CO2 emissions still grow by 21% compared to 2015 in the high ambition scenario. This is almost only half the growth (45% less) seen in the current ambition scenario. Carbon emissions from surface freight transport (road, rail and inland waterways) decrease only slightly (by 2%) between 2015 and 2050. Regional differences are great, however: In Europe, freight emissions decline by nearly 50% and over 40% in OECD countries while there is a 20% increase in Asia and more than 150% in Africa.
Table 5.3. Change in freight volumes and CO2 emissions in different scenarios
Percentage change compared to 2015
2030 |
2050 |
|||
---|---|---|---|---|
Scenarios |
Tonne-kilometres |
CO2 emissions |
Tonne-kilometres |
CO2 emissions |
Current ambition |
57 |
42 |
226 |
118 |
High ambition |
51 |
18 |
216 |
21 |
E-commerce |
61 |
45 |
238 |
127 |
3D printing |
52 |
33 |
135 |
59 |
New trade routes |
56 |
42 |
220 |
116 |
Energy transition for long distance heavy freight |
57 |
31 |
228 |
84 |
Autonomous trucks |
57 |
41 |
229 |
115 |
High capacity vehicles |
57 |
37 |
225 |
110 |
Logistic |
49 |
36 |
134 |
64 |
Technology |
51 |
13 |
220 |
22 |
Full disruptions |
49 |
13 |
133 |
-12 |
Sea and air freight volumes will see the largest increases by 2050. The associated CO2 emissions mean that overall freight emissions will still increase, if no additional policy measures are implemented. Air and maritime transport are intrinsically international and subject to often complex international agreements, while surface modes fall mostly under national or regional regulation. It is also assumed that efficiency gains will be lower for air and sea transport than for the surface modes (see Table 5.2).
Box 5.3. Updates to the modelling framework for freight transport
The framework for modelling freight was considerably updated for this Transport Outlook. Most significantly, the ITF international freight model and the ITF surface freight model where integrated into a single model. Currently international and domestic flows are aligned to match the national tonne-km activity forecast calibrated from the data reported by countries. Both are matched using a calibration procedure that improves the route assignment but also assesses the domestic component of international freight and the share of urban freight. The international component still estimates activity for 19 commodities for all major transport modes and routes, while taking into account different transport and economic policy measures (e.g. the development of new infrastructure networks, or the alleviation of trade barriers). OECD trade projections are used to convert trade in value terms into freight volumes. The model consists of the following components: 1.Trade flow disaggregation model, 2.Value-to-weight model, 3.Mode choice model and 4. Equilibrium route choice model. The main changes are:
1. A greater degree of disaggregation: The model now has 404 centroids, with a greater degree of resolution in Central Asia and Africa.
2. Incorporation of cost: A cost function of each mode and country or region was integrated into the model for improve estimations for the base year (2015) but also the sensitiveness of the model to policies and disruption that may affect freight costs (either in mode choice and route choice).
3. Route choice model: The model now includes a route choice model in the assignment step that generates maritime movements, the potential ports and transhipment locations to connect each pair of centroids. The probability of each alternative is calculated as a cost function of handling and transport costs (fuel and time) of each connection. This is integrated in an equilibrium assignment procedure that updates the probabilities of each route choice for each iteration.
4. Correct surface flows and modal split for countries: The surface freight volumes for each country are estimated based on the economic forecast. These estimates are converted into local flows and are assigned to the freight network. Each country is represented by a set of surface freight centroid, which identifies all the GDP concentrations of the country located at least 100 km apart using a set coverage optimisation model. A shortest path assignment is estimated between national surface freight centroids and the estimated tonne-km is converted into tonnes travelling between centroids estimating an average distance and proportionally to the GDP concentration and population of each centroid (gravity based model). Modal split of internal flows within the countries are produced with a logit model choice model with the cost of each mode to perform a connection between centroids as utility function. The initial assignment produces a layer of traffic that constraints the equilibrium assignment to the network of international freight volumes.
5. The ability to analyse the effect of policies or market exogenous factors that can be disruptive in the sector. The model steps and countries specific cost functions were adapted to be able to accommodate disruptive changes (technological, demand and supply structure) on the freight sector and estimate the potential reaction of freight volumes and related externalities.
Figure 5.4. Projected freight volumes by mode, 2030-50
Figure 5.5 and Figure 5.6 present modelled transport flows for 2015 and the activity at ports and airports.
Figure 5.5. Freight flows for sea, road, rail and inland waterways networks in 2015

Figure 5.6. Volume of activity at ports and airports in 2015

E-commerce
E-commerce can be defined as “the sale or purchase of goods or services, conducted over computer networks by methods specifically designed for the purpose of receiving or placing orders” (OECD, 2011[18]). Online sales occur either between businesses (B2B) or between businesses and consumers (B2C). This analysis focuses on B2C e-commerce activity; the fastest growing form of commerce and arguably the one with the greatest implications for transport, even if B2B transactions account for a greater total value.1
Initially operational in 1990, the World Wide Web was made freely available to the public in 1993. The rise of e-commerce began following the opening of the Web for commercial use in 1995. It was boosted by the introduction of internet browsers designed for non-technical users. By 1999, the global value of e-commerce sales had already reached USD 150 billion. It has continued to grow rapidly in the years since, powered by increasing internet connectivity, expanding global trade, and more sophisticated shipping technology. In 2017, the total value of global e-commerce sales was estimated at USD 2.3 trillion, an increase of 24.8% on the previous year (eMarketer, 2018[19]). E-commerce (both B2B and B2C) represented about 10% of global commerce in 2017.
Developing countries now account for the largest portion of new e-commerce, as its growth in developed countries is beginning to level off (UNCTAD, 2015[20]). Figure 5.7 shows the percentage of individuals who engaged in e-commerce activity across European countries in 2017 and Figure 5.8 shows steady growth in this rate in the European Union and selected developed countries.
The implications of the growing e-commerce industry for the transport sector were recognised as early as 2001 (OECD/ECMT, 2001[21]; OECD, 2003[22]). Almost twenty years later, the impact of e-commerce on transport patterns is undeniable. Nearly 80% of road freight experts surveyed by the ITF identified e-commerce as the trend most likely to be present in the sector by 2030 (ITF, 2018[6]). Respondents also indicated that they expect major e-commerce retailers to play an increasingly dominant role as logistics services providers in future years.
Figure 5.7. Share of population who made an online purchase in 2017 by country
Figure 5.8. Evolution of the share of population who made an online purchase, 2006‑17
What drives the development of e-commerce?
Online purchasing is associated with a number of socio-demographic characteristics, although the evidence is somewhat mixed. Some studies show that online purchasing activity is correlated with a high education level, an above average household income, as well as being female and Caucasians (Wang and Zhou, 2015[24]). Others found that men and younger people reported more online shopping activity than women and older citizens (Sener and Reeder, 2012[25]). Alternatively, other evidence suggests that there is greater interest in online purchasing among the elderly, disabled, two-worker households, and single parents (Mokhtarian, 2004[26]).
Given the great variety of goods and services available online, however, these mixed results are not surprising - the factors that affect the propensity and frequency of online purchasing activity are likely to vary according to what is being purchased. On the supply side, the determinants of a firm’s decision to sell online can include its size, membership in an industry group, and having a consumer (vs. business) clientele (Coad and Duch-Brown, 2017[27]).
The most important reasons for buying online reported by European Union residents in the year 2000 were access to products not otherwise available in a consumer’s area, price considerations, and the convenience of delivery options relative to in-store shopping. Factors that discouraged people from online shopping included concerns about after-sale service, the privacy of personal data, and delivery issues (European Commission, 2000[28]). Today, frequent online shoppers cite price and convenience as the most important reasons for shopping online. More recently, the most frequently cited reason for not shopping online more often is that customers enjoy having an in-store experience as well as being able to take the product home right away (Civic Consulting, 2011[29]), which indicates a continuing role for brick-and-mortar stores even as e-commerce continues to grow.
At a more aggregate scale, the pace of growth in e-commerce is also affected by business conditions (e.g. regulatory and tax environments) and technological developments. Goods delivery, payment systems, high-speed broadband availability, and retailer engagement have also been identified as factors explaining national differences in e-commerce activity (Civic Consulting, 2011[29]), with cultural factors and social norms also playing a role (Ben-Elia, Lyons and Mokhtarian, 2018[30]). This is particularly relevant in China, where online shopping has taken on a particular social significance and people now spend an average of 30 minutes a day shopping online (BCG, 2017[31]).
Concurrent trends in other domains are also expected to have a significant impact on e-commerce. The continued expansion of internet connectivity and the rise of mobile phone use across the globe are the most important. Transactions via mobile phones made up 58.9% of digital sales in 2017 and represent the fastest-growing purchase mode for e-sales (eMarketer, 2018[19]). Technological progress in areas such as the Internet of Things, autonomous vehicles and drones as well as artificial intelligence have also been highlighted as growth drivers for e-commerce (WEF, 2017[32]). In an “Internet of Things” world, for example, connected household devices could automatically re-order products when necessary. Advances in autonomous vehicles and drones could change transport patterns in the last mile of delivery, while artificial intelligence will, in turn, play an important role in the development of autonomous vehicles.
What are the implications of e-commerce for the transport sector?
The potential impacts of e-commerce on transport are highly complex. Identifying cause and effect in this domain is tricky and quantifying the precise impacts of e-commerce on transport demand and related CO2 emissions fraught with difficulties. Their direction and magnitude will depend on factors such as urban density, mode shares and energy mix, for example, as well as on the nexus of physical, psychological and socio-demographic factors noted above (Cullinane, 2009[33]; Kos-Łabędowicz and Urbanek, 2017[34]; Mokhtarian, 2009[35]; van Loon et al., 2014[36]). Determining the ways in which e-commerce could reshape transport ultimately requires considering of how online shopping will change consumer behaviour, with regard to both travel behaviour as well as behaviour that has implications for freight demand. More on-demand deliveries within narrow time windows will reduce vehicle payloads, and customer returns of e-commerce products mean rising delivery vehicle-kilometres and lower average loads.
These behavioural changes can produce three different types of aggregate impacts (Mokhtarian, 2004[26]). Firstly, e-commerce can shift the way in which consumers purchase goods without changing the volume or total value of the goods they buy. Secondly, the generally lower prices made possible by online shopping in principle enable consumers to buy more goods without spending more than they used to. Thirdly, the option to purchase online may increase the total amount that people spend on goods by inducing new demand and increasing per capita consumption.
Empirical evidence suggests that overall greater e-commerce activity adds net transport demand. The proprietary nature of freight data make comprehensive analyses of the impact of e-commerce on freight demand difficult, a number of studies have nonetheless investigated this question, most found that e-commerce has a positive impact on freight demand, although the magnitude of this impact varies (Bonilla, 2016[37]; Mangiaracina et al., 2015[38]; Zanni and Bristow, 2010[39]). With respect to passenger demand, the evidence suggests that, while B2C e-commerce can have both complementary and substitutive effects on passenger travel demand, most of the literature points to a complementary effect, i.e. an increase in net passenger transport demand. Overall, thus, the dominant view in the research literature holds that e-commerce has a complementary impact on personal travel, rather than substitutive one (Commons, 2009[40]; Hauptbibliothek et al., 2015[41]; Mokhtarian, 2009[35]; Wang and Lo, 2007[42]).2 The extent to which e-commerce changes current transport patterns will also depend on any mitigation measures put in place to accommodate this increased demand (see Box 5.2.)
E-commerce sales worldwide are projected to reach an average of 40% of the global market share in 2026, though this should vary by sector (WEF, 2017[32]). Governments worldwide have recognised the potential role of e-commerce in economic growth and have begun to actively promote it (UNCTAD, 2018[43]). The EU, for example, has launched initiatives to boost e-commerce, which including targets for the number of customers buying products online within and across member state borders (European Commission, 2013[44]; European Commission, 2016[45]). E-commerce will therefore almost certainly continue to put upward pressure on transport demand. The distribution of this demand over time, mode, demographic segment, and space, however, will depend on a variety of factors. The most disruptive effects are likely to be felt in the “last mile” due to increased volumes of activity and fragmentation of consignments.
E-commerce increases transport volumes and emissions
E-commerce is already changing logistics and is likely to play an increasingly dominant role in the way people obtain goods. This brings some decarbonising opportunities. But if unchecked, it is more likely to increase both emissions and congestion in cities. New business models offering free return of goods and requiring ever tighter delivery windows, constrain efforts to optimise operations and decrease the use of available capacity. Lower transport and transaction costs can lead to demand growth.
Policy can shape these developments. Promoting the use of collection points, off-peak deliveries and zero emission zoning will contribute to mitigating emissions. Other policies –e.g. distance-based charges - could nudge distribution operators to better use vehicle capacity and limit practices that foster less efficient transport and more congestion.
E-commerce is the only simulated freight disruption that actually drives up CO2 emissions, with a 4% increase of total CO2 emissions by 2050 compared to the current ambition scenario. This is directly related with increase in volume of activity (in t-km) that is associated with a strong growth of e-commerce.
Not all modes are affected in the same way, and different adoption rates are expected in different regions. The shares of different commodity types also changes over time. These factors combined help to explain why aviation movements increase more than any other mode, with road freight displaying the second largest increase. A disruptive impact of e-commerce will be particularly felt in urban operations and deliveries. These are the two most carbon intensive transport modes, so emissions are especially impacted by activity growth for these modes.
The increases seen in the emission projections do not account for likely losses of efficiency and average load for urban deliveries. There is no indisputable evidence for this, and some argue that increased economies of scale could even improve efficiency. But expert opinion tends towards assuming a negative impact on logistic efficiency, and hence more emissions and congestion. Thus, the negative impacts of e-commerce can potentially be even higher.
Table 5.4. Projected change in global freight volumes by mode in an e-commerce scenario, 2030 and 2050
Percentage change compared to current ambition scenario
Year |
Sea |
Air |
Road |
Rail |
Inland waterways |
Freight transport |
---|---|---|---|---|---|---|
2030 |
2 |
3 |
3 |
3 |
3 |
2 |
2050 |
3 |
11 |
6 |
4 |
2 |
4 |
Figure 5.9. Projected shifts of transport flows in the e-commerce scenario by 2050

3D printing
Three-dimensional (3D) printing could dramatically disrupt the current goods manufacturing processes and accompanying global trade patterns if it is scaled up sufficiently. Traditional manufacturing processes typically assemble materials produced at different locations. In contrast, 3D printing uses an additive process whereby items are produced through the progressive addition of very small layers of material to create the final product.
The ability to produce any desired form or shape anywhere could in principle render superfluous the shipping of semi-finished products to assembly plants. Equally, small-scale 3D printing could enable households to print certain consumer goods at home, vitiating the need for shipment. While 3D printing is still an emerging technology today, it thus has the potential to fundamentally transform many production processes and disrupt the demand for freight transport (Campbell et al., 2011[46]).
To date, 3D printing is mainly used to produce prototypes and for niche applications. It is used in the manufacture of industrial tools and their parts (such as jigs and fixtures), geometrically complex and lightweight products in the aerospace industry, prototypes of parts and tools in the automotive industry, polymer-based consumer products as well as some medical and dental devices (ING, 2017[47]; McKinnon, 2011[48]).
3D printing is gradually assuming a greater role in the manufacture of industrial components and machine tools, however. The number of 3D printers sold worldwide doubled between 2005 and 2011, and in 2017 sales of industrial 3D printing systems costing more than USD 5 000 rose by 80% on the previous year alone (MGI, 2012[49]; Wohlers Associates, 2018[50]). Companies spent more than USD 6 billion on 3D printers and related services in 2016 (ING, 2017[47]; Wohlers Associates, 2018[50]).
What drives the uptake of 3D printing?
As the cost of 3D printers and related materials is falling, the industry is set to further expand rapidly. The future evolution of 3D printing technology will also depend on the pace of innovation, including improvements in quality, the ability to print larger-size items, and the speed of printing. The unit cost of 3D-printed items is currently high relative to goods that are batch-produced in traditional factories. 3D printers are also still limited in the range and size of products they can produce. These aspects and other technical difficulties related to current 3D printing technologies constitute the major barriers to massive consumer adoption of 3D printing in households (Mckinnon, 2016[51]; OECD, 2017[52]).
The drivers for a widespread uptake of 3D printing by businesses are generally the same as for households. The costs of purchasing and maintaining industrial 3D printers, their longevity, and the ease of integrating them into existing production processes determine their attractiveness for businesses. The costs of 3D printing materials and of transporting these materials will also play a role.
What are the potential impacts of 3D printing on transport?
The distinction between industrial and consumer 3D printing is important when considering the potential disruptive impact of 3D printing on transport. The possible impacts of additive manufacturing on trade and urban freight movements could be much more significant if home production became the norm for a broad range of household products (Mckinnon, 2016[51]). Despite the ostensible benefits that may accompany increased 3D printing activity, experts disagree on the direction and magnitude of net impacts (Boon and van Wee, 2018[53]).
The additive nature of 3D printing offers several advantages over the subtractive nature of conventional manufacturing. Additive manufacturing may require less material than traditional manufacturing, produce less waste, and enable the production of commodities closer to the site of their final use. 3D printing could therefore reduce freight transport demand by consolidating material transport and manufacturing activities. Indeed, freight transport activity could be significantly reduced by delivering only 3D printing materials to the point of production through simple supply chains rather than producing parts at different locations and combining them through complex multi-link supply chains (Mckinnon, 2016[51]). Instead of products delivered at home in separately delivered packages, the materials of these products could be held in stocks and delivered in bulk to the final destination. As a result, the tonne-kilometres of freight moved in urban areas could be substantially reduced.
Domestic 3D printing would use less material for most products than conventional factory assembly, and so could also reduce the need to transport goods between factories. In a world of mass 3D printing, freight traffic per value unit of consumption could drop sharply, leading to lower costs, congestion and CO2 emissions. If 3D printing costs decreased significantly, this might lead to substantial re-shoring of manufacturing from countries with low labour costs in the Far East to Europe and North America (McKinnon, 2018[54]). Recent estimates suggest that 3D printing could make up as much as 50% of manufacturing activity, and that this would reduce world trade by 38% by 2040. According to these estimates, automotive, industrial machinery and consumer products would be the industries most affected and cross border trade in their commodities would decrease significantly (ING, 2017[47]).
Recent studies have challenged the view that 3D printing would shift manufacturing away from centralised factories to regional production sites or even consumer homes, however. They point out that 3D printers still mostly print parts rather than whole products, and that therefore most of the 3D products still need to be assembled in factories. Material for 3D printers will also still need to be shipped to factories or households.
Similarly, there are ground to question the argument that 3D printing can eliminate waste and avoid overproduction. It is true stocks of mass-produced goods are held in warehouses to be available in time for the predicted demand, while warehousing and the associated costs could in principle be avoided with 3D printing. However, unsold goods account only for 5% of most sectors’ revenues on average and thus have only a marginal impact on global freight movements.
Two of the most-touted sustainability benefits of 3D printing may thus be overstated (OECD, 2017[52]). Transport constitutes only a small share of the total environmental impact of any product, and the potential of 3D printing for reducing the global carbon footprint of freight therefore seems to be rather limited. Existing 3D printing technologies in any case remain limited for now to the fabrication of parts rather than entire products, and these parts still require transport to their assembly points as well as shipping to their final destinations (OECD, 2017[52]).
Depending on the extent of its uptake, 3D printing has significant potential to impact on the manufacturing industry and on global supply chains. The adoption of 3D printing on an unforeseen scale would imply significant changes to logistics and manufacturing as production processes would shift from away from centralised factories and closer to consumers. Given the current state and uptake of 3D printing, however, it seems unrealistic that it will significantly disrupt transport and logistics systems. While it is likely that 3D printing will expand into more industries, its uptake will be limited not least by its inability to compete with conventional production methods that can produce larger numbers of a given product at lower costs. 3D printing will probably increase its role in prototype production and the manufacture of small items, but is less likely to reach the scale of mass manufacturing unless its costs decrease significantly (OECD, 2017[52]). In a survey of road freight experts, the majority of respondents expressed the view that 3D printing would have no significant impacts on the sector (ITF, 2018[6]).
In a scenario with relatively favourable yet not disruptive assumptions, 3D printing equipment would account for 8% of total manufacturing equipment in 2040 (Westerweel, Basten and Fransoo, 2018[55]). In the simulation for this Transport Outlook, we assumed the most disruptive assumptions that suggest a 38% decrease in global trade. Most of this projected change is driven by a reduction in movement of high-value commodities that are today produced in the Far East and then shipped to Europe and North America. To the extent that 3D-printed products could become important components in the construction of low-carbon technologies such as electric vehicles, 3D printing could conceivably contribute to lowering the cost of these technologies and thus accelerate their market penetration.
Mass adoption of 3D printing could significantly reduce international freight volumes
Growing freight demand is the number one driver for increased CO2 emissions. These will not be reduced in a meaningful way unless demand growth stays substantially below current forecasts. Exogenous influences can have a significant impact on transport volumes and thus play a critical role in emissions reduction.
Among all simulated freight disruptions, 3D printing delivers the highest impacts on freight emissions, with a 27% decrease in freight CO2 by 2050 compared to the current ambition scenario. The main reason is a 28% decrease in transport volumes, mostly in electronics and other manufactured goods. Air freight declines more sharply relative to other modes, since with more 3D printing lighter, high-value goods are manufactured closer to consumption centres. Average loads tend to be higher due to a relative increase of heavier goods being moved, resulting in and overall increase in energy efficiency of freight transport.
Massive changes in logistical global supply chains occur if the most disruptive assumptions of 3D printing that can be found in the literature are simulated. Figure 5.10 shows that the ports and airports most active in moving manufactured goods would suffer the greatest declines. Hence, East Asia would see the sharpest fall in freight flows. Globally there would be a significant decrease in congestion and excess capacity across transport networks and at their main nodes, at least compared to the current ambition scenario.
Table 5.5. Projected change in global freight volumes by mode in a 3D printing scenario, 2030 and 2050
Compared to current ambition scenario, percentages
Year |
Sea |
Air |
Road |
Rail |
Inland waterways |
Freight transport |
---|---|---|---|---|---|---|
2030 |
-3 |
-24 |
-4 |
-3 |
-4 |
-3 |
2050 |
-32 |
-56 |
-15 |
-10 |
-26 |
-28 |
Figure 5.10. Projected shifts of transport flows in the 3D printing scenario by 2050

New international trade routes
Shifts in international trade routes could considerably alter freight transport demand in the coming years. These shifts could result from new and improved freight networks in Eurasia and Africa and from new maritime routes opening up in Arctic waters. Surface freight networks in North America are not expected to change significantly in the coming years. In South America, investment in infrastructure as a percentage of GDP remains low, and a corruption scandal involving the region’s largest construction company has halted many projects. Significant improvements in infrastructure in South America are therefore likely to be slow in the near future.
New canals could also provide alternative maritime routes that would shorten existing trade routes. The Kra Canal across the Malayan peninsula would cut the distance for oil from the Middle East to reach China and Japan by 1 200 km, the equivalent of two to three days.3 The proposed Nicaragua Canal across the Central American isthmus could provide an alternative to the Panama Canal that would be better able to accommodate the biggest ships. Both projects seem unlikely to materialise in the near future, however.
Regular train connections already carry some freight between Europe and China via the Russian Federation. Three main railway corridors have been identified that span the Eurasian continent to connect China, Central Asia, Europe, South East Asia and South Asia. Among these corridors, the northern route – using the Trans-Siberian railways or Kazakhstan’s rail system – is currently the only route with stable and reliable transport services and infrastructure (UIC, 2017[56]). It consequently has the highest traffic volumes.
Only about 1% of trade between Europe and Asia is transported via rail, while more than 90% is transported via ships (UIC, 2017[56]). But rail freight flows between East Asia and the European Union have increased significantly in recent years, from 25 000 Twenty-foot Equivalent Units (TEU) in 2014 to 145 000 TEU in 2016, which is still significantly less than the containers transported by sea between Asia and Europe. Finally, Azerbaijan, Kazakhstan, Georgia and Turkey have agreed to construct the Trans-Caspian International Transport Route (TITR) as part an intermodal East-West transport infrastructure initiative.
In Africa, investment in infrastructure projects is accelerating quickly due to the recognition of their importance for the development of the continent (AfDB/OECD/UNDP, 2017[57]). A number of initiatives seek to increase regional integration in Africa, including the Boosting Intra-Africa Trade action plan of the African Union and the Trade Facilitation Agreement of the World Trade Organisation (WTO). Road, rail, and maritime transport have been targeted for improvement. Freight connectivity on the continent is currently highest in South Africa. Greater connectivity between South Africa and eastern Africa is expected to be developed by 2030, and between eastern and western Africa by 2040.
With regard to new maritime routes in the Arctic, the Northeast and Northwest Passages already experience some use during the ice-free summer period. The Transpolar Sea Route is navigable throughout the year, but only with powerful icebreakers. Increasing melting ice cover over the Arctic Sea has created new possibilities for commercial shipping. The Northern Sea Route, for example, is predicted to be ice-free on a seasonal basis sometime between 2040 and 2050 (Smith and Stephenson, 2013[58]). The Russian Federal Agency for Maritime and River Transport has reported a volume of 9.7 million tonnes of goods shipped on the Northern Sea Route in 2017 (Marine Insight, 2018[59]), compared to 2 million tonnes throughout most of the first decade of the 2000s. Although, this represents an infinitesimal amount of intercontinental trade and the main maritime trade routes.
What could drive changes in international trade routes?
Investment in infrastructure is the most significant driver of the development of new freight routes in Eurasia and Africa. In China, the major expansion of rail connectivity with Europe has gathered political momentum and considerable political will exists to increase network capacity. Although transport by rail is five times more expensive than transport by sea, it is about 1.7 times faster. This makes rail an attractive mode for transporting highly time-sensitive goods, such as fashion goods, electronics, car parts and perishables including food. The significant increase in rail freight flows between East Asia and the EU in recent years can be attributed to reductions in transit times and an increase in reliability, which are in turn a result of better infrastructure as well as more efficient handling, customs and border-crossing processes.
In Africa, regional integration has become a priority and investment in infrastructure projects is increasing. In 2015, member states of the Common Market for the Eastern and Southern Africa, the Eastern African Community and the Southern Africa Development Community signed a tripartite trade agreement to enhance market integration, infrastructure development and industrialisation. Under the African Union’s Programme for Infrastructure Development in Africa, many rail, road, and maritime investment projects are planned or under way.
In the Arctic, maritime operators who are considering using the Northern Sea Route face a trade-off between the gains from shorter distance and the higher costs of Arctic shipping. Apart from meteorological conditions and heightened safety concerns in Arctic waters, operators face logistical barriers due to scarce infrastructure, strict certification requirements, and tight environmental regulations, e.g. voyage planning restrictions aiming to protect marine ecosystems (USCG, 2017[60]). The Polar Code sets strict standards including on ship design, crew training, fuel tank characteristics, or sewage discharge. Even more stringent environmental regulations could apply to Arctic shipping in the future, for instance regarding the use of heavy fuel oil, already prohibited in the Antarctic. Conforming to these regulations reduces the net economic gains of shorter transit times. While the Northern Sea Route could still be an economically viable option under specific circumstances – mostly for bulk shipping from the Russian Arctic – the market potential for other types of shipments remains highly uncertain (Kiiski, 2017[61]; USCG, 2017[60]). Should the Arctic become reliably ice-free at some future date, however, this could increase the likelihood of more Arctic shipping.
What are the implications of trade route changes for international freight transport?
The expansion of rail cargo transport between China and Europe has gathered momentum. Rail freight flows between East Asia and the EU are expected to increase at an annual growth rate of 14% (UIC, 2017[56]). Modernising infrastructure and improving the efficiency of customs processes at border crossings could shorten transit times by four to seven days on Euro-Asian Transport Linkage Routes. In Africa, the impacts of increased infrastructure investment are beginning to materialise. The construction of the Mombasa-Kampala corridor between Kenya and Uganda, for example, reduced transit times from fifteen to five days (OECD, 2011[18]). In Namibia and Zambia, the Walvis Bay Corridor Group has reduced the Southern African Development Community clearance time from forty-eight to two hours.
Using the Northern Sea Route for maritime freight between Northern Europe and Japan could reduce voyage distances by 37% relative to routing through the Suez Canal (Buixadé Farré et al., 2014[62]). Distance from Northern European ports to Korea would be reduced by 31%, to China by 23% and to Chinese Taipei by 17% (Bekkers, Francois and Rojas‐Romagosa, 2018[63]). Regular use of the North-West Passage could reduce voyage distance between North America and large ports located in Northeast Asia by up to 20% (Ørts Hansen et al., 2016[64]). For trade between South Asian countries and southern European countries, however, the conventional route via the Malacca Straits and the Suez Canal remains shorter. Melia, Haines and Hawkins (2016[65]) model future reductions in sea ice and find that future transit between Europe and Asia will be reduced by ten days by 2050, and by 13 days in subsequent years. Routes between Asia and North America only stand to save four days because the route through the Panama Canal is relatively short.
Box 5.4. Enhancing freight connectivity in Central Asia
Freight connectivity is fundamental to increasing the competitiveness of countries and promotes economic growth, social integration and development. Improved freight connectivity can benefit countries and regions by providing peripheral areas better access to domestic and international markets for trade, reducing costs for the domestic economy through improved infrastructure and services, relieving congestion and augmenting revenues from expanded transit.
Central Asia is characterised by relatively poor connectivity, despite its historical role as a land bridge between Asia and Europe. The freight volumes passing through the region between Asia and Europe are currently less than 2% of what is carried by sea. The region lags behind others on several dimensions of connectivity and integration, which hinders the development of trade. Economic integration in the area is limited by a low density of settlement and economic activity, infrastructure bottlenecks, ageing road and rail networks, long distances to major markets and to sea ports, as well as numerous regulatory and policy barriers to cross-border flows.
In the context of an OECD project funded by Kazakhstan, the International Transport Forum (ITF) reviewed freight connectivity in Central Asia with a focus on Kazakhstan, Kyrgyzstan, Mongolia, Tajikistan and Uzbekistan. The assessment included a) an analysis of the current level of connectivity in the region with regard to the needs of the regions’ economies and the efficiency of freight and logistics networks; b) a review of countries’ transport and logistics strategies including infrastructure investments plans; and c) identification of possible future bottlenecks and missing links under alternative trade and policy scenarios.
The results showed that current investment plans in the region will improve connectivity but are likely insufficient to accommodate future trade growth. To prepare for future growth and to improve connectivity, the Central Asian countries have developed national infrastructure plans and are participating in programmes such as the Central Asia Regional Economic Cooperation (CAREC), the Transport Corridor Europe-Caucasus-Asia (TRACECA) and China’s Belt and Road initiative which aim to develop international economic and transport corridors.
Quantitative analysis based on the ITF international freight model showed, however, that even with implementation of the planned infrastructure projects capacity on many links will be insufficient to accommodate all future freight flows. As Figure 5.11 shows, demand for infrastructure capacity increases by 2030 on both international corridors as well as regional connections. Existing infrastructure plans focus on key international corridors, yet ensuring local business are connected to the main corridors is crucial for realising the benefits from agglomeration economies.
Figure 5.11. Projected capacity needs for road and rail freight transport, 2030

Reducing the strain on capacity requires infrastructure improvements, such as the construction of new lanes and refurbishment of existing ones, as well as efficiency improvements (e.g. use of high capacity vehicles, development of consolidation centres, or electrification of rail lines).
Other measures can also improve regional connectivity, such as improving border crossing facilitation to reduce waiting times. Refining the national and regional logistics strategies as well as enhancing institutional capacity for making evidence-based decisions and planning under uncertainty. Strengthened regional and international cooperation will also contribute to shorten travel times and make them more predictable, lowering the costs for moving goods along international corridors. Further insights can be found in ITF (Forthcoming[66])
Although they shorten transit distances, new maritime routes through the Arctic may not reduce the climate-related impacts of shipping activities in a significant way. This is mostly due to the difficult sailing conditions in the Arctic and the implications this has for fuel efficiency. In open waters, ships are not required to change their speed very often and can optimise the engine load and fuel efficiency. In the Arctic, this is not the case. Often adverse weather and shallow seas require frequent changes in speed in direction, often making engine optimisation impossible. Highly variable engine loads reduce fuel efficiency and generate up to 50% more black carbon (Lack and Corbett, 2012[67]), the negative effects of which are exacerbated when emitted in the Arctic (Yumashev et al., 2017[68]).
More maritime freight via the Arctic could increase trade volumes and shift emission-intensive production in Northeast Asia. As a result, the potential gains permitted by shorter transit distances in the Arctic may be more than offset by the negative emissions impacts associated with this activity (Bekkers, Francois and Rojas‐Romagosa, 2018[63]; Lindstad, Bright and Strømman, 2016[69]).
Rail freight rates between China and Europe have dropped from thirteen to five times the rates of maritime transport (Merk, 2016[70]). This gap between rail and sea freight rate could continue to narrow if the cost increases of the 2020 sulphur emissions cap can be reflected in maritime transit prices and ocean freight rates pick up. Substantial subsidies from Chinese regional governments to Eurasian rail services, on the order of USD 2 000‑2 500 per TEU, could at some point be phased out, which would also diminish the financial viability of rail freight in the region (Rail Freight, 2017[71]).
Cooperation with Russia will be crucial in ensuring continued freight flows through the region, since the current Trans-Siberian and Baikal-Amur rail links on the Russian rail network are suffering from capacity shortages, which has limited the growth of rail transit volume through Russia (Global Risk Insight, 2017[72]). The implementation of more stringent regulations on emissions and pollutants from maritime freight could also result in a shift towards rail freight. Regular operation of container block trains constitutes the most competitive, logistics market-oriented model for enhanced operationalisation Euro-Asian Transport Links (EATL) inland routes. Even if freight volumes on the inland routes will never approach those on maritime links, they can be increasingly used for high-value and time-sensitive freight. In Africa, trans-continental freight transport options could lead to increased intra-African trade and could also shorten international trade routes by 2050, if current and planned transit infrastructure projects in Africa continue to reap similar benefits in terms of cost and time savings.
With respect to the potential for increased maritime freight transit in the Arctic, it is estimated that by 2050 the entire Arctic coastline and most of the Arctic Ocean will experience an additional 60 days of open water each year on average, including a range of areas that will experience more than 100 days additional days of open water (Barnhart et al., 2016[73]). Favourable meteorological conditions and infrastructure and technical solutions could conceivably even allow year-round operations by 2030 (Bekkers, Francois and Rojas‐Romagosa, 2018[63]), though uncertainty around the first ice-free year in the Arctic is estimated to be about 20 years (Jahn et al., 2016[74]; Notz and Stroeve, 2016[75]).
As a result, the Russian Federal Agency for Maritime and River Transport expects a six-fold increase in the volume of maritime freight in the Arctic over the next three years. China has included the Northern Sea Route in the scope of its Belt and Road Initiative since June 2017 and released a white paper outlining its plans for a “Polar Silk Road” in the Arctic in 2018 (The State Council Information Office of the People’s Republic of China, 2018[76]). Despite indications of steady growth of sea traffic on the Northern Sea Route, however, a significant amount of uncertainty remains with regard to future sailing conditions in the Arctic and the cost-effectiveness of this transit. Given this uncertainty and the likelihood of negative climate impacts of black carbon emissions, the potential for new maritime routes in the Arctic to contribute to significant decreases in CO2 emissions appears limited at this time. Furthermore, increasing cargo transport by rail could displace some of the anticipated rise in maritime traffic in the Arctic region.
Despite the ostensible advantages with respect to distance and transit time, substantial economic risks of maritime travel through the Arctic remain, including reductions in fuel efficiency, uncertainty with respect to arrival dates, and safety-related risks for their crew and vessels. These factors can erode any economic savings from transit via Arctic routes and will prevent their more rapid adoption. Some evidence suggests that the Northern Sea Route will be profitable for ordinary merchant ships only by 2040 (Ørts Hansen et al., 2016[64]). Nonetheless, registered traffic had a five-fold increase on the Northern Sea Route in the past decade, and depending on the evolution of climate conditions and infrastructure investments this can become a viable option in the future.
New trade routes will not affect emissions significantly, but can change the face of transport networks
New trade routes will have no significant impact on CO2 emissions from freight or global freight volumes, according to the simulation results for this Transport Outlook. A shift in trade routes would reduce transport volumes by 2% (3% for seaborne freight) and emissions by 1% in 2050, compared to the current ambition scenario.
There would nonetheless be very important impacts on global logistic chains and transport networks. The Mediterranean Sea and the Indian Ocean would see a drop in freight traffic of one fifth (21% and 19% respectively) by 2050 compared to current ambition scenario, whereas traffic in the Artic that is currently marginal would increase exponentially surpassing volumes in the South Pacific or Caribbean. Ports along the Suez route between East Asia and Northern Europe would lose traffic compared to the current ambition scenario. Those located in strategic positions along the new Artic route would see significant increases in this simulation, with Busan in Korea registering the largest increase, above 50% compared to the current ambition scenario. Figure 5.12 depicts the projected percentage change in tonnes of freight transport flows and loaded/unloaded cargo in 2050 in a new trade routes scenario compared to the current ambition scenario.
The projected shift in transport flows would also impact the surface transport networks that provide access to the ports. In China and Europe especially, inland flows adjust to changing patterns in maritime routes. This can lead to extra capacity along routes that lose traffic and to congestion in segments where traffic will grow.
In Eurasia, connectivity improvements associated with existing initiatives and other projects leads to an increase in rail volumes compared to the current ambition scenario – although not at the scale of regular shipping in the Artic. The results also show a consolidation of flows on the rail lines that will be improved. In Central Asia, better access to oceanic ports can also produce visible changes in the routes used to access the sea from this region.
Simulation of aggressive disruption of trade routes (employing the specifications shown in Table 5.7) shows the impact of significant infrastructure improvements – namely rail, but also road - along Eurasia and opening up the Artic to commercial shipping without impediments from Europe to East Asia and East Coast of America to East Asia (the latter still with higher costs than average). How likely this will actually be is a matter discussed above and not yet clear.
Figure 5.12. Shift in transport flows in the new trade routes scenario by 2050

Energy transition for long-distance road freight
Road freight’s contribution towards freight CO2 emissions is sizeable and its relevance for efforts to decarbonise the transport sector as a whole is increasing. A number of decarbonisation strategies have been identified, including improving fuel efficiency and mainstreaming the use of alternative energy sources. Important near-term mitigation measures comprise efficiency improvements such as aerodynamic retrofits, reduced tire rolling resistance, vehicle weight reduction, enhanced engine efficiency and hybridisation. Ultimately, decarbonising road freight will hinge on a transition to ultra-low and zero-emission technologies.
This transition will be particularly challenging given the weight of heavy duty vehicles (HDVs) and the long distances they need to cover. Electric road systems (ERS) and hydrogen fuel cells currently represent the most feasible technologies for mitigating road freight emissions while meeting the energy demands of the sector. However, progress in battery technology such as super-fast chargers and battery swapping systems, could radically change the trajectory of the energy transition in road freight. Breakthroughs in low-carbon liquid fuels should also not be ruled out. Even if not foreseeable at present, advanced biofuels or synthetic renewable fuels (“e-fuels”) could come to play a role, as well as an accelerated deployment of carbon capture and sequestration (CCS).
Electric roads systems enable moving vehicles to receive electricity via overhead catenary, ground conductive or inductive technologies. Conductive overhead catenary systems transmit energy via a pantograph mounted on a vehicle’s roof, similar to electric trains, trams and trolleybuses. The underlying technology has been in use for more than 130 years and can be integrated and operated within the existing road infrastructure. Conductive rail systems transmit energy to rails in the ground and then to the vehicle via a slide-in current collector system.
Finally, inductive charging systems transmit energy from the road to the moving vehicle wirelessly via a magnetic field, requiring the installation of coils that generate an electromagnetic field in the road as well as receiving coils for electricity generation in the vehicle. This requires no mechanical contact for transmitting electricity. Vehicles that operate on ERS must also be equipped with autonomous energy sources such as batteries or hydrogen fuel cells that power the engine when driving on non-ERS roads. In the case of ERS-compatible battery electric vehicles (BEVs), batteries can be recharged while traveling on an electric road system. ERS represent a promising method for powering long-haul heavy goods transport, but will not be practical for heavy duty vehicles servicing smaller and less frequently used collector roads.
Hydrogen fuel cell and battery technologies could be used as a complement to electric roads in the regions or trip legs that are not covered by ERS infrastructure. The most common method of producing hydrogen is through a steam reforming process that uses fossil fuels such as natural gas. Hydrogen can also be produced from the electrolysis of water using electricity, although with low efficiency levels. Hydrogen is stored in fuel cells and converted to electricity to power movement.
What drives the transition to renewable energy in long distance road freight?
An important factor in the development of electric road systems and hydrogen technologies for powering road freight transport are the costs associated with their uptake. To make them an attractive option and facilitate uptake at scale, the infrastructure costs of alternative fuels could not be borne solely by fleet owners in order for it to be attractive enough to adopt.
Several estimates suggest that electric road systems are characterised by lower total costs than other alternative fuels (Cambridge Econometrics, 2018[77]; Connolly, 2017[78]; Kasten et al., 2016[79]; Siemens, 2017[80]). The cost of ERS will depend on the technology used (catenary overhead, conductive rail or inductive charging) and the autonomous drivetrain type of the heavy duty vehicles (i.e. whether the vehicles can remain in electric-drive mode on roads not equipped with ERS), for how long, and whether the vehicle battery can be recharged at charging points. Table 5.6 provides an overview of cost estimates for the different ERS technologies. Cost estimates for electrifying vehicle drivetrains depend on the reference vehicle, the type of electrification that is envisaged (hybrid or full battery electric vehicle), the battery system that defines the electric drive range of the vehicle (i.e. the distance the vehicle can run on battery power when being outside of an ERS), and the costs for allowing the vehicle to be dynamically charged via an ERS. Battery costs and the cost of hybridisation and electrification are expected to decrease significantly in the future as battery technology advances and economies of scale manifest themselves.
Table 5.6. Cost estimates for electric road systems
Type of system |
Infrastructure costs(1) (million EUR/km) |
Vehicle costs(2) (EUR) |
Infrastructure maintenance costs (% of investment costs) |
---|---|---|---|
Overhead catenary |
2.2 |
+50 000 (in 2020) +19 000 (in 2050) |
2.5 |
1.5 |
+50% |
— |
|
1.5‑2.5 |
+40 000 (in 2020) +25 000 (in 2030) +15 000 (in 2040) |
4.0 |
|
1.6(3) (0.8 in long run) |
|||
0.7‑2.0 |
+5 000 (compared to a hybrid heavy duty vehicle) |
— |
|
Conductive rail |
0.4(4) |
— |
— |
Inductive charging |
>3.1 |
— |
— |
Note: (1) Per kilometre of two-lane highway, fitted on existing road infrastructure. Includes costs for electrical wiring, rails and poles, connections to the electric grid, substations with transformers, control units, related civil works etc. (2) Compared to a conventional heavy duty vehicle unless stated otherwise. Includes costs to hybridise or fully electrify the drivetrain of the vehicle. (3) Provided in USD for one lane. (4) Assuming that 20 000km of roads in Sweden are electrified.
Source: Kasten et al. (2016[79]); Jancovici, Schuller and Borie (2017[81]); CCGD (2017[82]); IEA (2017[9]); Viktoria Swedish ICT (2013[83]); eRoadArlanda (2018[84]).
Under current economic and technological conditions, overhead catenary systems are probably the most cost-effective system (Jancovici, Schuller and Borie, 2017[81]; Kasten et al., 2016[79]). Although the total infrastructure costs for batteries, hydrogen and electric road systems would be similar, ERS is likely to be least costly over time for an equivalent number of zero-emission vehicles in service (Kasten et al., 2016[79]). Operational costs will also depend on the share of the vehicle mileage on ERS-equipped roads, the price difference between the conventional fuels (i.e. diesel) and electricity, and the subsidies, preferential policies or tolls that may be applied in certain regions.
None of these systems are currently in widespread use. Much of the discussion regarding the cost-benefit comparison of alternatives is based on assumptions of future developments that are highly uncertain. A survey carried out by the ITF found that road freight experts see the lack of existing infrastructure, difficulty in scaling up production, and high vehicle costs as the greatest barriers to the adoption of electric road systems (ITF, 2018[6]).
As ERS are not a practical solution for all parts of the road network, hydrogen fuel cell technology could play a role in mitigating emissions from non-ERS road freight travel. Hydrogen is a relatively energy-dense fuel compared to current battery technologies. This gives hydrogen fuel cell electric vehicles (FCEVs) greater autonomy than plug-in electric vehicles (PEVs), as well as more cargo space. Although the tank-to-wheel (TTW) efficiency of hydrogen fuel cell technology is high, the production of this fuel is relatively inefficient compared to electricity generation. Low overall energy efficiency, high costs for vehicle, network and infrastructure, as well as the challenge of scaling up production are among the barriers to greater uptake of FCEVs (ITF, 2018[6]).
Considerable uncertainty surrounds the magnitude of the cost reductions associated with fuel cell systems in the future, particularly with respect to the coextensive developments in battery technologies. Financial tools may either encourage the uptake of these technologies (e.g. purchase subsidies) but may also be used to recover the costs associated with infrastructure construction and maintenance.
Box 5.5. Low-carbon fuels
Liquid fuels remain prevalent in transport thanks to their relatively high energy density, portability, storage stability, and ease of delivery. This is the case in particular for high-mileage heavy duty vehicles for cargo transport. The extensive distribution infrastructures in place give liquid fuels an additional advantage. Alternative energy sources for road freight transport such as electricity or fuel cell require more investment to deploy and a longer time scale to achieve a meaningful market share.
Among the technologies capable of reducing the carbon footprint of road freight and delivering climate-friendly mobility in terms of lifecycle emissions are low-carbon intensity crude oil, upstream and refinery carbon capture and storage/utilisation, advanced biofuels and drop-in low-carbon fuels derived from water and CO2.
Even if alternative energy sources for mobility such as electricity are making rapid advances, the vast majority of global mobility demand will continue to rely on burning fossil fuels for decades. Crude oil production, transport, and refining into finished products account for as much as 15‑40% of fuel well-to-wheel greenhouse gas emissions.
While in use around the world for quite some time, biofuels still make up a small portion of total fuel consumption in most regions. Only in very few countries do they have a significant share of the fuel use, among them in Brazil and the United States. Most biofuels are crop-based (e.g. sugar cane, corn or vegetable oil), which raises concerns regarding indirect land use change.
A dramatic increase in the use of crop-based biofuels would mean a substitution of food crops and large-scale conversion of arable land - unless mitigated by yield-intensive crop practices and land use policies (Macedo et al., 2012[85]; Nepstad et al., 2014[86]). The carbon emissions originating in these land use changes can offset any emission savings potential from biofuels (Valin et al., 2015[87]).
Where biofuels can be produced from waste, algae or cellulose they become a much more attractive option for decarbonising transport, although such non-crop solutions have proven difficult to scale up. Both crop and non-crop biofuels that involve a fermentation process can offer even lower lifecycle GHG reductions if coupled with CCS.
The caveats do not disqualify biofuels from the set of available CO2 mitigation solutions for road transport. The impacts of indirect land use change can vary greatly from country to country, for instance. The case of Brazil demonstrates how the widespread adoption of Ethanol produced from sugar cane can contribute to lower emissions even when the total life cycle and land use changes are taken into account (La Rovere, Pereira and Simões, 2011[88]; Rothkopf, 2008[89]; Schroeder, 2010[90]). This being said, conditions in Brazil (a climate suitable for sugar cane, large amounts of arable land and a well-developed agro-industrial complex) cannot be easily reproduced at global level.
This highlights an important insight: the pathways to decarbonising transport should be adapted to the specific conditions of different regions, as solutions that may have a high emissions reduction potential and convincing economic case and in one place might not be applicable to others.
The growing awareness of the problems associated with land-use change has spawned more and more interest in the scientific community for alternative biofuels. Among these are synthetic fuels made from water and CO2 as primary inputs. The principle of synthesising fuel is hydrogen generation via electrolysis or direct water splitting, and reacting it with CO2 into molecules which are blended with the fossil base fuel, to reduce overall carbon intensity. If the electricity required for the synthesis is from renewable sources and the carbon atoms obtained through reducing CO2 (e.g. from captured combustion or captured from the air), the synthetic fuel is nearly carbon neutral. Gradually over time, the proportion of synthetic fuel blended into the fossil base could increase and increase the mitigation effect. A co-benefit of synthetic fuels is combustion is substantially cleaner than fossil fuels in terms of nitrogen oxides (NOx) and soot emissions.
What are the impacts of transitioning to alternative fuels in long-distance road freight?
Battery electric vehicles and vehicles on electric roads do not generate tailpipe emissions. The same is true for and fuel cell electric vehicles (FCEV), yet lifecycle greenhouse gas emissions from FCEVs have been estimated at twice those of diesel trucks, at least with the current electricity mix in Germany (Kühnel, Hacker and Wolf, 2018[91]). The well-to-wheel energy efficiency of heavy duty vehicles on electric road systems have been estimated at 77%, compared to 62% for battery electric trucks and 29% for hydrogen trucks (Kasten et al., 2016[79]; Moultak, Lutsey and Hall, 2017[92]). Among ERS technologies, overhead catenary systems are considered to have the greatest energy efficiency. Furthermore, Overall emissions reductions of ERS will depend on the degree of electrification of heavy duty vehicles, the system’s overall energy efficiency and the share of travel on non-ERS equipped roads.
In terms of the impact of ERS on the electricity grid, ERS is expected to be less demanding than more conventional battery vehicles. This is because the continuous electricity supply via the ERS allows for smoother load profiles on the electricity network. The energy storage capacity made possible by hydrogen fuel can help to foster and maximize the use of electricity produced from renewable sources.
Decoupling road freight transport from CO2 emissions will require significant investments. Substantial financial commitments will be required from private companies, for instance the vehicle manufactures that will have to adapt their production. Significant funding will also be required from the public sector, notably for the creation of distribution and fuelling/charging networks. All three types of electric road systems are currently being tested, mainly in Germany, Sweden, France, China, Japan and Korea (eRoadArlanda, 2018[84]; Heise, 2017[93]; Jacob and Caso Florez, 2018[94]; Scania, 2016[95]; Transport & Mobility Leuven, 2017[12]). These tests will be useful to identify viable business models, regulatory requirements and international standards. They will also help to address safety concerns. New players from outside transport might become important partners, for instance utility companies. These could help shape the business models for electric road systems or charging station networks.
In many countries, the great majority kilometres driven by heavy duty vehicles are concentrated on relatively small portion of the road network (Kasten et al., 2016[79]). Thus, new infrastructure for alternative energy sources will most likely be implemented on trunk routes with a high volumes of freight traffic, for instance between distribution centres, ports or rail terminals. Here, electric road systems may be financially viable for private operators within as little as five years (Schulte and Ny, 2018[96]). Here, they will also generate the greatest CO2 reductions. A network effect could emerge after 2030, especially if framework policies such as strict emission standards for heavy duty vehicles put sufficient pressure on vehicle manufacturers and operators. Because road freight trips are often international, a successful deployment of electric road systems will also require concerted international efforts to ensure inter-operability across borders.
With respect to hydrogen, some public money is already being invested in hydrogen re-fuelling stations. These are now accessible in a number of cities worldwide. Projections of the future uptake of fuel-cell electric vehicles are limited, despite the substantial cost reduction potential of hydrogen technologies (US DOE, 2017[97]). The reason are the investment risks of infrastructure development (IEA, 2017[98]). If battery technology for heavy trucks on long-haul operations advances significantly, battery-electric vehicles could supplant electric road systems and fuel cell electric vehicles as the most promising CO2 mitigation technology for road freight.
In sum, the large-scale deployment of electric road systems and hydrogen over the next decade appears unlikely. In the absence of a significant scaling-up of current efforts, ERS and hydrogen fuel cell technologies are only likely to become mainstream in the mid- to long-term, perhaps between 2030 and 2050. The deployment of ERS and heavy duty vehicles powered by hydrogen fuel cells will vary across countries and regions, depending on the (cost) attractiveness of alternatives to mitigate CO2 emissions, the state of the electric grid, the road system, and the political will to achieve climate goals.
It is also highly unlikely that a single technology will be able to replace diesel or gasoline internal combustion engines. There is a degree of complementarity between different solutions: vehicles powered by a direct supply of electricity through catenaries can also have batteries, fuel cells or combustion engines running on biofuels. Options that require large-scale infrastructure investments might not be available in some regions, or be less cost-effective there. Achieving total, or near-total, decarbonisation will require a range of solutions that are complementary and can be adapted to different contexts.
Energy transition for long-distance road freight is necessary to decarbonise road freight
Non-urban road freight currently accounts for 87% of road transport volume and 77% of its emissions. It contributes 43% of freight transport emissions, more than any other mode. Its share of total transport CO2 emissions, including those of passenger transport is 16% of emissions.
Thus, efforts to decarbonise transport will fail without a successful energy transition for long-haul road freight. Of the disruptions evaluated for this Transport Outlook, replacing fossil fuel as the main energy source for long-haul road freight achieves the second-highest impact on freight emissions, with a 16% decrease in CO2 emissions by 2050 compared to the current ambition scenario.
The impact of an energy transition in long-distance will vary across world regions. The differences are explained by varying adoption rates assumed and the shares of road in surface transport in each region. Europe will thus see bigger decreases in emissions from this transition, because it has a high adoption rate and high modal share for road. North America will see smaller gains because the share of road is smaller and hence changing its energy source has less impact on emissions.
Figure 5.13. Projected CO2 emissions from surface modes in the energy transition scenario by region, 2030-50
Figure 5.14. Projected road and rail freight transport volumes in the energy transition scenario, 2030-50
An energy transition also influences transport costs. These will be higher initially but then gradually fall below those of current internal combustion engines, due to lower fuel and maintenance costs as well as the abatement of infrastructure costs. Again, there will be variations by region.
A shift towards low- or zero-carbon fuels also impacts demand and modal share. Because costs are already lower there, road will gain modal share from rail in Europe by 2030, but not in other regions. By 2050, with wider deployment and falling costs across the world, a more general shift from rail to road takes place, particularly in China and India. This assumes, however, that there will not be equivalent cost decreases or other counter-acting initiatives from the rail sector.
Autonomous Trucks
Road freight is particularly suited to full automation. Self-driving trucks would enable significant savings on labour costs, which currently comprise a third or more of the operating costs in Europe and North America. There is thus a strong commercial incentive to introduce automated trucking.
Most of the long-haul heavy duty operations in developed countries take place on highways where automation is easier to implement than in highly complex urban traffic settings. Not least, driverless trucks will be able to operate 24 hours per day, thus optimising asset use, making it easier to avoid peak hours and providing greater flexibility in fleet management.
Important barriers to driverless trucks exist nonetheless. Specifically, more progress is needed in vehicle-to-everything (V2X) communication and in standardisation.
Most experts agree that driverless trucks will become a reality in ten to twenty years, albeit only on specific highway corridors between logistic centres with large volumes of demand. Road freight is ahead of other sectors in this field, but other markets also show great potential like public transport where several trials have taken place and services are already operating in cities (ITF, 2018[99]).
Besides individual self-driving trucks, convoys of semi-automated vehicle convoys linked via vehicle-to-vehicle communication systems (“platoons”) are in advanced tests.
What drives the uptake of autonomous trucks?
The significant cost reductions and increases in operational efficiency provide strong incentives for the industry to develop and deploy autonomous trucking. The way and speed with which automation will be adopted depends on a host of factors. Some technological advances are still needed. The current infrastructure will need to be adapted. Clarity is needed on the exact business model. Questions regarding insurance and liability require answers. Public concerns about safety and security risks must be answered – and those answers might drive up costs and limit the commercial attractiveness of driverless trucks.
Wide spread adoption of automated road freight vehicles and operations needs common standards across the business, or at least on sufficiently large markets. The regulatory framework thus plays a critical role, as it conditions to which extent the industry will be able to enjoy the benefits of automated road freight. Presently, a degree of uncertainty persists on how permissive or restrictive the regulatory regimes will ultimately be, these developments are associated with the safety, security and congestion impacts of this technology (something further discussed in Chapter 3). Nonetheless, as noted above, automation applied to trucks moving along highways not passing through highly congested urban areas at this stage seems to have fewer obstacles and clearer benefits than for individual passenger-vehicles.
Also presently uncertain is the level of automation that can be attained in road freight. It is not clear that fully driverless trucks (i.e. level 5 automation according to the standard classification of SAE International, see Chapter 3) will be possible for all road freight operations. Yet it is certain that vehicles will be equipped with systems that will increasingly assist drivers. Such lower levels of automation can already increase fuel efficiency, improve routing and change the current role of truck drivers. All of which can still contribute to reduce operational costs.
What are the implications of autonomous trucks use for transport systems?
Autonomous trucks and truck platooning can bring significant cost savings. In an expert survey carried out by the ITF, a majority of respondents estimates the cost reduction potential of platooning at 10% or more. Half of the respondents saw an even greater cost-savings potential for fully autonomous vehicles, which they put at greater than 25%. At this point, savings would reach the level of labour costs, which make up between 25% and 45% of the cost of road freight operators. While estimated savings are still at the lower end of the cost scale, operators would also reap indirect benefits, notably the ability to use their trucks more flexibly since mandatory rest times for drivers would no longer be required. Not least, self-driving trucks would provide an answer to the shortage of professional drivers faced by the haulage industry (ITF, 2017[100]).
Truck platooning can decrease the wind drag of vehicles driving closely packed in a column and thereby increase fuel efficiency. But its benefits are more associated with the reduction of operational costs. The contribution of autonomous trucks towards decarbonisation is less clear. They might increase driving efficiency, allow for higher loads and avoid congestion by using off-peak periods. But large cost reductions can lead to higher demand, thus more transport activity and, more emissions rather than less.
More than 50% of experts surveyed by ITF on this question believe that truck platooning will be in general use by 2030 and autonomous vehicles by 2050. There have been already several trials with digitally connected truck platoons (Dutch Ministry of Infrastructure and the Environment, CEDR and RDW, 2016[101]) and autonomous vehicles are already in operation in very delimited and controlled environments like ports and mines. Nonetheless, there are still open questions about the extent to which both these systems will eventually be deployed. As of today, there are no commercial operations with fully driverless trucks even on highways, although some trials with a driver on board have taken place (Davies, 2017[102]).
In view of the uncertainties still surrounding the deployment of fully autonomous trucks, this technology was not included it in the modelling of any baseline.
For a disruptive scenario we assume that the fuel efficiency gains of fully automated road freight vehicles are in line with the most optimistic expectations in the surveyed literature, resulting in a 14% reduction of carbon emission per tonne-kilometre. The operational costs of self-driving trucks per tonne-kilometre are 45% lower than current values, due to lower (or zero) labour cost and higher operational efficiency. In this disruptive scenario, all regions see the use of automated trucks grow more quickly on inter-urban trips than in urban environments. Like with the parameter variations on other scenarios the adoption rates follow a logistic curve to the 2050 target year that reaches a target value by 2050.
Autonomous trucks have moderate impacts on emissions
Overall global CO2 emissions will not change in a relevant order of magnitude as a result of shifting road freight from conventional to autonomous trucks. There is a slight decrease of 1% by 2050 compared to the current ambition scenario. Significantly lower road costs bring down overall transport costs which slightly increase global transport volumes by 1%. They also induce a shift from rail and inland waterways to road. Transport by air and sea are less affected by this change.
Though there is an emissions decrease from surface modes for most regions there are exceptions, like for North America (see Figure 5.15). This region sees a 15% drop in rail volumes, whereas road freight increases by 18% compared to the current ambition scenario. Among the potential disruption of road freight transport examined in this chapter, autonomous trucks cause the most drastic mode shift towards road and away from rail and inland waterways, more so than HCVs or zero to low-emission trucks (see Figure 5.14, Figure 5.16 and Figure 5.19). Even if autonomous vehicles should be more efficient than current trucks, they will still be more carbon intensive than rail. Hence the emissions increase in North America.
Figure 5.15. Projected CO2 emissions from surface modes by region in the autonomous truck scenario, 2030-50
Figure 5.16. Projected road and rail freight transport volumes in the autonomous truck scenario, 2030-50
The significant potential of autonomous trucks to cut costs allows higher returns, which can in turn be reinvested in increased efficiency and alternative fuel technologies. Thus, automation can indirectly help to overcome the high initial cost barrier of some of these technologies. On the other hand, a steep decrease in costs that make road transport more competitive can also spur a rise in demand that could offset any efficiency gains. Policy can address this dilemma, for instance by imposing stringent emission standards for automated trucks and ensuring they operate in off-peak periods to avoid congestion.
High capacity vehicles
The weight and dimensions of road freight vehicles are nationally regulated. In most countries, heavy goods vehicles are considered to be those weighing more than either 3.5 or 4.5 tonnes. High capacity vehicles (HCVs) are tucks that exceed the general weight and dimension limitations set by national regulations. They usually operate under special provisions within limited geographical areas or on specific routes. HCVs can move more cargo with fewer vehicle-kilometres driven, which results in lower fuel consumption per unit of transported cargo. This has implications for transport operators, cargo shippers, road freight regulators, consumers and the general public.
National standards mean that a 22-metre, 5-axle vehicle weighing 44 tonnes may be considered an HCV in one country but as a standard truck without access restrictions in another. A number of countries have either introduced HCVs permanently or are piloting them in order to explore the impacts that the use of larger and heavier vehicles can entail. Among these countries are Australia, Canada, the United States, Mexico, Argentina, New Zealand, South Africa and several countries in Europe
In the European Union (EU), the European Modular System (EMS) allows member states to choose combinations of existing standardised modules. The EMS therefore allows operators to exceed the general size and weight restrictions by combining smaller modules compliant with the regulation in place. This provision was initially intended to accommodate the larger trucks used in Finland and Sweden since the 1980s. The advantage of EMS is that it allows a high degree of flexibility in adapting vehicles to different situations. Operators have the ability to use longer combinations where possible and shorter combinations or single modules where regulations require it. Figure 5.17 shows how the EMS enables flexible operation of high capacity vehicles.
Figure 5.17. The European Modular System of trucks
What facilitates the proliferation of high capacity vehicles for road freight?
Operational, market and regulatory drivers are behind the uptake of high capacity vehicles (Aronietis et al., 2016[104]). Operational drivers consist of technology pushes and cost-saving measures. Technology pushes refer to the availability of technology, in this case vehicle modules. The cost-saving measures include the fuel- and labour-related costs that can be reduced per unit of cargo that is transported by high capacity vehicles.
The market drivers of HCV adoption promote cost savings through the competition-induced pressure towards higher performance in road freight operations. Regulatory drivers include applicable government regulations for road freight transport, such as safety- and efficiency-related regulations. Typical policy goals include reducing the number and impact of crashes, improving environmental performance and increasing operational efficiency. The presence of all three types of drivers indicates that the adoption of high capacity vehicles may be quite fast. The main barrier is the lack of a regulatory framework in some regions.
What are the impacts of high capacity vehicles on road freight transport?
Longer and heavier trucks will increase transport costs per truck by 5‑12%. Yet 10‑50% fewer vehicles are required to transport the same amount of cargo. Thus transport costs per unit of load actually decreases (Vierth et al., 2008[105]). As a secondary effect, such lower haulage costs can in turn shift freight activity away from rail or inland waterways towards road. It may even stimulate additional demand for road freight transport. They can also make it more difficult for less CO2-intensive but more costly freight modes to win market share. The induced demand effect is unlikely to cancel out the effect of fewer total-vehicle kilometres (OECD, 2011[106]). The effect of HCVs on mode split seems to have been overestimated in prior research (de Jong, 2017[107]).
Indeed, mode shift is only one possible reaction to the reduced costs generated by greater efficiency in road freight transport. Other responses from operators may include changes to the logistics network related to depot locations, shipment size, the consolidation of freight, and reduction of empty driving. They can also include changes to transport demand such as the use of different suppliers, marketing to different customers, or selecting different production locations. In an example from Sweden, increases in road tonne-kilometres originated mainly in other factors than increased efficiency due to the utilisation of HCVs (Vierth, 2017[108]).
The evidence in the literature on the impacts of HCVs has been mixed (Christidis and Leduc, 2009[109]). This in large part due to the varying assumptions on road freight price elasticity and the specific payloads, distances, and costs considered. Empirical assessments of the known impacts are largely positive (OECD, 2011[106]; McKinnon, 2014[110]). In Canada, Sweden and Australia the introduction of HCVs went along with reduced road traffic and CO2 emissions, for example (Vierth et al., 2008[105]; Woodroffe, 2017[111]). The overall changes caused by introducing HCVs will depend on a number of factors such as the adoption rate, geography of the region, operational patterns of HCV operators, type and density of the cargo, and the networks of competing transport modes.
Capacity increases in truck size beyond 60 tonnes payloads and 25.25 metres length are likely to yield additional environmental benefits. In one study, simulations indicated that further increasing the weight and length restrictions on road freight vehicles in Sweden from 64 tonnes and 25.25 metres to 74 tonnes and 34 metres would decrease CO2 emissions by up to 12.17 mega-tonnes between 2018 and 2058 (Pålsson et al., 2017[112]). In Finland, the impact of introducing increased weight and height limits of up 76 tonnes and 4.4 metres for road freight vehicles (up from 60 tonnes and 4.2 metres) is estimated to have led to a reduction of 65 000 tonnes of CO2 emissions in 2015 (Liimatainen and Nykänen, 2016[113]).
The challenge for operators using high capacity vehicles is to optimise vehicle loading. Depending on the cargo type, capacity will be limited either by the specified weight restrictions or by the volume of goods the vehicle is able to carry. Therefore an increase in weight limits will mostly affect dense cargo that tends to limited by its weight, such as steel. An increase in the maximum allowed dimensions of the vehicle will mostly impact the transport of cargo that is limited by volume, such as textiles or footwear.
Emissions produced by diesel-powered road freight vehicles continue to have a detrimental impact on air quality, despite improvements following the introduction of EURO emission and safety classes. Reducing the harmful emissions from diesel fuel combustion is technologically difficult, though. Thus, no further reduction of local emissions from diesel vehicles class is expected in the foreseeable future. Technologies that do not use hydrocarbon fuels, such as electric vehicles, are vastly more promising in the long-term for tackling local pollution and greenhouse gas emissions (see the discussion on e-highways and energy transition above). Although high capacity vehicles primarily offer near-term benefits for reducing CO2 emissions, they have potential in applications in specific contexts, such as in North America, where US and Canadian vehicles are characterised by radically different environmental performance (Figure 5.18).
Figure 5.18. CO2 emissions of alternative heavy goods vehicles in North America
Using 0.037 litres of fuel and emitting 98.79 grammes of CO2 per cargo-unit, Canadian B-trains are 68% more efficient than US tractor semitrailers, which use 0.063 litres of fuel and emit 165.9 grammes of CO2 per cargo unit (Woodroffe, 2017[111]). Only the relaxation of existing limits on vehicle size and weight will enable greater uptake of high capacity vehicles limits. Another barrier to the uptake of HCVs is the potential costs associated with updates of existing road infrastructure. These may be needed to accommodate the increased weight and dimensions of high capacity vehicles. To the extent that HCVs reduce traffic volumes, they can conceivably also extend the lifespan of reinforced infrastructure, however (Pålsson et al., 2017[112]).
Stringent enforcement of HCV regulation thanks to the opportunities offered by GPS tracking, automated weighing or vehicle measuring technologies can help to reduce adoption barriers. They can reliably ensure that the allowed weights and dimensions are not exceeded, and that the vehicles stay on specific routes or within designated geographical areas. Since HCVs have specific infrastructure requirements, for instance minimum roundabout radii or maximum load for bridges, it makes sense to limit HCVs to specific parts of the road network and update relevant infrastructure elements on those. Ideally, the areas of operation would be situated where niche transport markets would benefit from efficiency increases to bring higher value to society.
High capacity vehicles have moderate impacts in emissions and modal shift
Simulation results show that the gains in increased average load and logistic efficiency of high capacity vehicles more than make up for the increased volume of activity and overall emissions decrease. This is the case even if the modal shift from rail (and inland waterways) to road is taken into account. Nonetheless, minimising this reverse modal shift and induced demand that results from lower costs of road transport is critical, as without it this alternative might not contribute towards decarbonisation.
Overall, the high capacity vehicles disruption scenario displays a very moderate decrease of 3% in the CO2 emissions of HCVs compared to the current ambition scenario. Still, this is a more significant decrease than in the autonomous trucks scenarios. The impact on total freight volumes is reduced, although there is a marginal increase in the share of road freight transport compared to rail, but much lower than for the scenario with disruption by autonomous trucks.
Changes in costs were not concentrated in the specific routes where HCVs would operate, but on the wider network of highways and non-urban roads. On the dedicated routes where these types of trucks operate on a large scale the impacts will be magnified, even if the aggregate impacts are moderate.
Figure 5.19. Projected surface freight transport volumes in the high capacity vehicle scenario, 2030-50
Figure 5.20. Projected CO2 emissions of surface freight modes in the high capacity vehicle scenario, 2030-50
Disruptive scenarios in freight transport
Freight-related emissions are estimated to fall 12% below 2015 levels by 2050 in the full disruption scenario, where all the potential disruptions described above are combined and policies are set to target high ambitions in terms of logistical and technical efficiency. Without disruptions and external shocks, policy-induced if needed, it is unlikely that freight emissions can be significantly reduced.
Only applying all logistic and technology levers in combination with the disruptive deployment of energy transition of long distance road freight and the occurrence of exogenous shocks such as a wide scale adoption of 3D printing - that will considerably decrease the growth in trade values - are emission reductions achieved.
Such a scenario is highly disruptive, massively changing transport costs, activity volumes, logistic chains and with new technologies in wide spread use. Although demand still grows in this scenario, its annual compounded growth rate from 2015 to 2050 is 2.5% compared to 3.4% in the current ambition scenario. Transport flows across the networks decrease, although with a few exceptions where new trade routes develop, notably Artic shipping and improved rail lines in Eurasia.
The shifts that take place in this scenario require new infrastructure, for instance, new investments will be needed to allow for an energy transition. At the same time, there may be reduction in freight flows at certain corridors resulting in overcapacity in some links and nodes of the network (e.g. ports and airports). This underlines the relevance of investment planning and project appraisal in the transport sector, incorporating as much as possible risk analysis and uncertainty. It also points to the paramount importance of data both to have a better understanding of current dynamics and the potential impacts of future developments.
International trade by air and sea will see the sharpest decreases in activity compared to our current ambition scenario. Inland waterways would also have significant losses since it is closely associated with maritime shipping. Road and rail would lose the least, particularly road, which although decreasing is still able to capture some traffic from rail and inland waterways.
Table 5.7 describes the assumptions of disruptive and non-disruptive development pathways for each of the potential disruptions discussed above. Table 5.8 specifies how these alternative pathways are combined in three alternative scenarios. In addition to a scenario in which all disruptions occur (full disruption scenario), the logistics scenario assumes that only exogenous and logistical disruptions occur, specifically e-commerce, 3D printing, a decrease in international transports of coal and oil, the opening of new trade routes, and improved logistical efficiency (or average loads) as employed in the high ambition scenario (based on the IEA’s EV30@30 scenario). The technology scenario assumes that disruptions of a technological nature occur, specifically autonomous trucks, high capacity vehicles and energy transition for heavy goods vehicles.
Figure 5.21. Shift in transport flows in the full disruption scenario by 2050

Table 5.7. Assumptions for disruptive scenarios for freight transport
Potential disruptions |
Assumptions for non-disruptive scenario |
Assumptions for disruptive scenario |
|||||
---|---|---|---|---|---|---|---|
|
E-commerce |
Urban freight sees additional 5% demand increase compared to current ambitions in more developed regions by 2050 |
Urban freight sees an additional 25% demand increase compared to current ambitions in more developed regions by 2050 and 10% on other regions. Inter-urban freight increases by a quarter of urban values. |
||||
|
3D printing |
No change from current uptake rate |
Decrease of 38% in overall value of trade by 2050 compared to international trade forecasts employed in current and high ambition scenarios. Differentiated decreases by commodity types. |
||||
|
New trade routes |
Planned improvements to infrastructure in central Asia region. Improved conditions for Europe-Asia connections through the Artic (but still with capacity and speed restrictions). |
Planned infrastructure improvements in Central Asia are implemented. Travel times improve, capacity increases and transport costs (rail) decrease on two corridors connecting East Asia to Europe. Border crossing times shorten along these routes. Regular shipping connections between Asia and Europe through the Artic exist by 2030. The Artic also opens to Asia to North American routes in 2030, but with higher costs than regular shipping connections. Infrastructure quality improves in Africa, with shorter travel times and lower costs. |
||||
|
Energy transition in long distance heavy freight |
Technological assumptions in line with the IEA-NPS (IEA, 2018a) |
37% of heavy truck t-km powered by alternative fuels by 2050. Costs are initially higher than for conventional fuels but become lower by 2050. Differences in uptake and costs exist by region. |
||||
|
Autonomous trucks |
No change from current uptake level |
Up to 90% uptake by 2050 on inter-urban routes in some regions (Europe, North America, China, Japan and South Korea). Uptake for urban freight is lower. Carbon intensity decreases 14% and costs 45% compared to current values. |
||||
|
High-capacity vehicles |
5% of inter-urban road freight transported with high capacity vehicles. Truck loads increase 50% and costs fall 20% per tonne-kilometre where HCVs are adopted. |
20% of inter-urban road freight transported with HCVs. Truck loads increase 50% and costs fall 20% per t-km where HCVs are adopted. |
Table 5.8. Disruptive scenarios in freight transport
Potentially disruptive developments |
|||||
---|---|---|---|---|---|
Trends/Disruptions |
Logistics |
Technology |
Full disruptions |
||
|
E-commerce |
Disruptive |
Non-disruptive |
Disruptive |
|
|
3D printing |
Disruptive |
Non-disruptive |
Disruptive |
|
|
New trade routes |
Disruptive |
Non-disruptive |
Disruptive |
|
|
Energy transition in long distance heavy freight |
Non-disruptive |
Disruptive |
Disruptive |
|
|
Autonomous trucks |
Non-disruptive |
Disruptive |
Disruptive |
|
|
High-capacity vehicles |
Non-disruptive |
Disruptive |
Disruptive |
|
Mitigation measures |
|||||
Trends/Disruptions |
Logistics |
Technology |
Full disruptions |
||
|
International coal and oil consumption |
Coal use decreases 50% by 2035 Oil use decreases 33% by 2035 |
Coal use decreases 50% by 2035 Oil use decreases 33% by 2035 |
Coal use decreases 50% by 2035 Oil use decreases 33% by 2035 |
|
|
Efficiency improvements and electric vehicles |
NPS-IEA |
EV30@30-IEA |
EV30@30-IEA |
|
|
Logistics efficiency |
EV30@30-IEA |
NPS-IEA |
EV30@30-IEA |
Note: Refer to Table 5.7 for definitions of Disruptive and Non-disruptive assumptions
An increase in logistical efficiency and exogenous shocks per se are not able to globally reduce CO2 emissions below 2015 levels by 2050. Yet they do curb the growth of freight transport activity significantly, by 28% to 2050 compared to the current ambition scenario, which contributes to reducing related carbon emissions by 25% compared to this baseline.
3D printing contributes most to reducing freight demand, and in the scenario that assumes this technology can be scaled up, the volume of international trade declines substantially compared to the current ambition scenario. Reduced movement of oil and coal also cuts back global trade volumes. Also adding to the decline, but less so, is the shortening of freight distances resulting from the new trade routes.
The reduction in transport flows is sharper for air and sea freight, modes more directly associated with international trade. In comparison with the current ambition scenario, transport volumes decline by 50% for air cargo and 35% for maritime freight. The major changes in transport activity volumes mentioned in the full disruptions scenarios have origin in the disruptions included in this logistic scenario.
Highly ambitious technological targets and related disruptions are able to curb emissions to a higher extent than logistical measures and exogenous shocks. Nonetheless, technology alone cannot reduce emissions by 2050 to levels equal or below 2015. In this scenario there is still a 22% increase in emissions from 2015 to 2050.
Besides overall emission decrease in line with the high ambition scenario, there are also important modal shifts, namely from rail and inland waterways towards road. The compounded effects of cost decreases of road freight due to a large-scale adoption of autonomous trucks, high capacity vehicles and low- or zero-emission trucks leads to a global 8% increase in road freight volumes compared to the current ambition scenario by 2050. Rail activity decreases by 12% and inland waterways by 2%.
Figure 5.22. Projected freight volumes by mode, 2030-50
Figure 5.23. Projected CO2 emissions from freight transport by mode, 2030-50
Regions with a relatively high share of rail freight tend to see greater modal shifts towards road. Rail activity in North America, for instance, suffers a 19% drop by 2050 compared to the current ambition scenario (Figure 5.24) while road freight volumes grow by 20%. China, India and Australia also see important increases in road share and decreases in rail activity. Air transport grows as well in this scenario, simply because there is a relative increase of higher value to density commodities that favour air transport.
Figure 5.24. Shift in transport flows in the technology scenario by 2050

References
[57] AfDB/OECD/UNDP (w2017), African Economic Outlook 2017: Entrepreneurship and Industrialisation, OECD Publishing, Paris, https://dx.doi.org/10.1787/aeo-2017-en.
[104] Aronietis, R. et al. (2016), “Forecasting port-level demand for LNG as a ship fuel: the case of the port of Antwerp”, Journal of Shipping and Trade, Vol. 1/1, p. 2, http://dx.doi.org/10.1186/s41072-016-0007-1.
[73] Barnhart, K. et al. (2016), “Mapping the future expansion of Arctic open water”, Nature Climate Change, Vol. 6/3, pp. 280-285, http://dx.doi.org/10.1038/nclimate2848.
[31] BCG (2017), What China Reveals About the Future of Shopping, https://www.bcg.com/publications/2017/retail-globalization-china-reveals-future-shopping.aspx (accessed on 1 February 2019).
[63] Bekkers, E., J. Francois and H. Rojas‐Romagosa (2018), “Melting ice Caps and the Economic Impact of Opening the Northern Sea Route”, The Economic Journal, Vol. 128/610, pp. 1095-1127, http://dx.doi.org/10.1111/ecoj.12460.
[30] Ben-Elia, E., G. Lyons and P. Mokhtarian (2018), “Epilogue: the new frontiers of behavioral research on the interrelationships between ICT, activities, time use and mobility”, Transportation, Vol. 45/2, pp. 479-497, http://dx.doi.org/10.1007/s11116-018-9871-x.
[37] Bonilla, D. (2016), “Urban vans, e-commerce and road freight transport”, Production Planning & Control, Vol. 27/6, pp. 433-442, http://dx.doi.org/10.1080/09537287.2016.1147093.
[53] Boon, W. and B. van Wee (2018), “Influence of 3D printing on transport: a theory and experts judgment based conceptual model”, Transport Reviews, Vol. 38/5, pp. 556-575, http://dx.doi.org/10.1080/01441647.2017.1370036.
[62] Buixadé Farré, A. et al. (2014), “Commercial Arctic shipping through the Northeast Passage: routes, resources, governance, technology, and infrastructure”, Polar Geography, Vol. 37/4, pp. 298-324, http://dx.doi.org/10.1080/1088937X.2014.965769.
[77] Cambridge Econometrics (2018), Trucking into a Greener Future, European Climate Foundation, https://europeanclimate.org/wp-content/uploads/2018/08/Trucking-into-a-Greener-Future_Final.pdf (accessed on 5 October 2018).
[46] Campbell, T. et al. (2011), Could 3D printing change the world? Technologies, potential, and implications of additive manufacturing, Atlantic Council, http://globaltrends.thedialogue.org/wp-content/uploads/2014/11/Could-3D-Printing-Change-the-World-Technologies-Potential-and-Implications-of-Additive-Manufacturing.pdf (accessed on 24 January 2019).
[82] CCGD (2017), Concept d’autoroute électrique: Évaluation socioéconomique, Analyse Théma, Commissariat Général au Développement Durable - Service de l’économie, de l’évaluation et de l’intégration du développement durable, https://www.idrrim.com/ressources/documents/9/5010-Thema-Concept-autoroute-electrique-.pdf (accessed on 21 January 2019).
[2] Château, J., R. Dellink and E. Lanzi (2014), “An Overview of the OECD ENV-Linkages Model: Version 3”, OECD Environment Working Papers, No. 65, OECD Publishing, Paris, https://dx.doi.org/10.1787/5jz2qck2b2vd-en.
[109] Christidis, P. and G. Leduc (2009), Longer and heavier vehicles for freight transport, European Comission.
[29] Civic Consulting (2011), Consumer market study on the functioning of e-commerce and Internet marketing and selling techniques in the retail of goods Final Report Part 1: Synthesis Report Prepared by Civic Consulting, http://www.civic-consulting.de/reports/study_ecommerce_goods_en.pdf (accessed on 1 February 2019).
[27] Coad, A. and N. Duch-Brown (2017), “Barriers to European Cross-border e-Commerce”, JRC Digital Economy Working Paper, https://ec.europa.eu/jrc/en/publication/eur-scientific-and-technical-research-reports/barriers-european-cross-border-e-commerce (accessed on 1 February 2019).
[40] Commons, T. (2009), The New Mobility Agenda, http://www.ecoplan.org/library/prospectus.pdf.
[78] Connolly, D. (2017), “Economic viability of electric roads compared to oil and batteries for all forms of road transport”, Energy Strategy Reviews, Vol. 18, pp. 235-249, http://dx.doi.org/10.1016/j.esr.2017.09.005.
[15] Crozet, Y. and A. Woodburn (2014), Development of rail freight in Europe: what regulation can and cannot do, Centre on Regulation in Europe (CERRE), Brussels, https://www.cerre.eu/publications/development-rail-freight-europe-what-regulation-can-and-cannot-do-0 (accessed on 12 October 2018).
[33] Cullinane, S. (2009), “From Bricks to Clicks: The Impact of Online Retailing on Transport and the Environment”, Transport Reviews, Vol. 29/6, pp. 759-776, http://dx.doi.org/10.1080/01441640902796364.
[102] Davies, A. (2017), Self-Driving Trucks Are Now Delivering Refridgerators, Wired, https://www.wired.com/story/embark-self-driving-truck-deliveries/ (accessed on 28 January 2019).
[107] de Jong, G. (2017), Research on the Estimation of Price Elasticities in Freight Transport, http://www.csrf.ac.uk/event/oecd-international-transport-forum-workshop-on-modal-shift/.
[101] Dutch Ministry of Infrastructure and the Environment, CEDR and RDW (2016), European Truck Platooning Challenge 2016 Lessons Learnt, Dutch Ministry of Infrastructure and the Environment, https://eutruckplatooning.com/PageByID.aspx?sectionID=131542&contentPageID=529927 (accessed on 2 October 2018).
[19] eMarketer (2018), Worldwide Retail and Ecommerce Sales: eMarketer’s Updated Forecast and New Mcommerce Estimates for 2016—2021, https://www.emarketer.com/Report/Worldwide-Retail-Ecommerce-Sales-eMarketers-Updated-Forecast-New-Mcommerce-Estimates-20162021/2002182 (accessed on 31 January 2019).
[84] eRoadArlanda (2018), Globally unique electrified road enables fossil-free road transport - eRoadArlanda, https://eroadarlanda.com/globally-unique-electrified-road-enables-fossil-free-road-transport/ (accessed on 21 January 2019).
[45] European Commission (2016), A comprehensive approach to stimulating cross-border e-commerce for Europe’s citizens and businesses, https://ec.europa.eu/transparency/regdoc/rep/1/2016/EN/1-2016-320-EN-F1-1.PDF (accessed on 4 February 2019).
[44] European Commission (2013), E-commerce Action Plan 2012-2015: State of Play 2013, http://ec.europa.eu/information_society/newsroom/image/document/2017-4/130423_report-ecommerce-action-plan_en_42073.pdf (accessed on 4 February 2019).
[16] European Commission (2011), Roadmap to a Single European Transport Area – Towards a competitive and resource efficient transport system, European Commission, Brussels, https://eur-lex.europa.eu/legal-content/EN/TXT/PDF/?uri=CELEX:52011DC0144&from=EN (accessed on 23 April 2019).
[28] European Commission (2000), Summary of the results of the Public Consultation on the future of electronic commerce in the Internal Market and the implementation of the Directive on electronic commerce (2000/31/EC), http://ec.europa.eu/information_society/newsroom/image/document/2017-4/consultation_summary_report_en_2010_42070.pdf (accessed on 1 February 2019).
[114] European Union (n.d.), Eurostat, https://ec.europa.eu/eurostat/web/transport/data/database (accessed on 16 August 2018).
[23] Eurostat (2019), Internet Purchases by Individuals, http://appsso.eurostat.ec.europa.eu/nui/show.do?dataset=isoc_ec_ibuy&lang=en (accessed on 29 April 2019).
[115] Eurostat (2002), ESA95 manual on government deficit and debt.
[72] Global Risk Insight (2017), China’s Belt and Road Initiative: Risk Insights, https://44s2n02i19u61od84f3rzjqx-wpengine.netdna-ssl.com/wp-content/uploads/2018/04/GRI-Chinas-Belt-and-Road-Risk-Insights.pdf (accessed on 22 January 2019).
[41] Hauptbibliothek, U. et al. (2015), “Impacts of home shopping on vehicle operations and greenhouse gas emissions: multi-year regional study Impacts of home shopping on vehicle operations and greenhouse gas emissions: multi-year regional study”, http://dx.doi.org/10.1080/13504509.2015.1124471.
[93] Heise (2017), eHighway: Bauarbeiten für Oberleitungen an hessischer Autobahn beginnen, https://www.heise.de/newsticker/meldung/eHighway-Bauarbeiten-fuer-Oberleitungen-an-hessischer-Autobahn-beginnen-3922190.html (accessed on 21 January 2019).
[8] Holguín-Veras, J. et al. (2016), “Direct impacts of off-hour deliveries on urban freight emissions”, Transportation Research Part D, http://dx.doi.org/10.1016/j.trd.2016.10.013.
[3] IEA (2018), Global EV Outlook 2018: Towards cross-modal electrification, International Energy Agency, Paris, https://dx.doi.org/10.1787/9789264302365-en.
[98] IEA (2017), Energy Technology Perspectives 2017: Catalysing Energy Technology Transformations, International Energy Agency, Paris, https://dx.doi.org/10.1787/energy_tech-2017-en.
[9] IEA (2017), The Future of Trucks Implications for energy and the environment Second edition, IEA, Paris, https://doi.org/10.1787/9789264279452-en (accessed on 12 October 2018).
[47] ING (2017), 3D printing: a threat to global trade, https://think.ing.com/uploads/reports/3D_printing_DEF_270917.pdf (accessed on 24 January 2019).
[1] ITF (2018), Decarbonising Maritime Transport Pathways to zero-carbon shipping by 2035, ITF, https://doi.org/10.1787/b1a7632c-en (accessed on 24 August 2018).
[99] ITF (2018), Safer Roads with Automated Vehicles?, OECD Publishing, Paris, https://doi.org/10.1787/b2881ccb-en.
[6] ITF (2018), Towards Road Freight Decarbonisation - Trends, Measures and Policies, ITF, Paris, http://www.itf-oecd.org (accessed on 21 January 2019).
[100] ITF (2017), Managing the Transition to Driverless Road Freight Transport, International Transport Forum, Paris, https://doi.org/10.1787/0f240722-en (accessed on 2 October 2018).
[66] ITF (Forthcoming), Enhancing Connectivity and Freight in Central Asia | ITF, ITF, Paris, https://www.itf-oecd.org/enhancing-connectivity-and-freight-central-asia (accessed on 15 March 2019).
[94] Jacob, B. and M. Caso Florez (2018), Electric Road Systems : PIARC Special Project, https://www.itf-oecd.org/electric-road-systems-piarc-special-project (accessed on 3 August 2018).
[74] Jahn, A. et al. (2016), “How predictable is the timing of a summer ice-free Arctic?”, Geophysical Research Letters, Vol. 43/17, pp. 9113-9120, http://dx.doi.org/10.1002/2016GL070067.
[81] Jancovici, J., A. Schuller and S. Borie (2017), L’Autoroute Électrique une Innovation pour Réduire les Émissiosn de CO2 du Transport de Marchandises, http://www.carbone4.com/wp-content/uploads/2017/02/201702_Autoroute-electrique_Communication_Carbone-4.pdf (accessed on 21 January 2019).
[79] Kasten, P. et al. (2016), Erarbeitung einer fachlichen Strategie zur Energieversorgung des Verkehrs bis zum Jahr 2050 Endbericht, https://www.umweltbundesamt.de/sites/default/files/medien/377/publikationen/2016-11-10_endbericht_energieversorgung_des_verkehrs_2050_final.pdf (accessed on 17 August 2018).
[61] Kiiski, T. (2017), Feasibility of Commercial Cargo Shipping Along the Northern Sea Route, 2017, http://www.utupub.fi/handle/10024/130546 (accessed on 22 January 2019).
[34] Kos-Łabędowicz, J. and A. Urbanek (2017), “Do Information and Communications Technologies influence transport demand? An exploratory study in the European Union”, Transportation Research Procedia, Vol. 25, pp. 2660-2676, http://dx.doi.org/10.1016/J.TRPRO.2017.05.156.
[91] Kühnel, S., F. Hacker and G. Wolf (2018), Oberleitungs-Lkw im Kontext weiterer Antriebs-und Energieversorgungsoptionen für den Straßengüterfernverkehr, Oko-Institut e.V., Berlin, https://www.oeko.de/publikationen/p-details/oberleitungs-lkw-im-kontext-weiterer-antriebs-und-energieversorgungsoptionen-fuer-den-strassengueterfe/ (accessed on 18 September 2018).
[88] La Rovere, E., A. Pereira and A. Simões (2011), “Biofuels and Sustainable Energy Development in Brazil”, World Development, Vol. 39/6, pp. 1026-1036, http://dx.doi.org/10.1016/J.WORLDDEV.2010.01.004.
[67] Lack, D. and J. Corbett (2012), “Black carbon from ships: a review of the effects of ship speed, fuel quality and exhaust gas scrubbing”, Atmos. Chem. Phys, Vol. 12, pp. 3985-4000, http://dx.doi.org/10.5194/acp-12-3985-2012.
[113] Liimatainen, H. and L. Nykänen (2016), Impacts of Increasing Maximum Truck Weight-Case Finland, http://www.tut.fi/verne/aineisto/LiimatainenNykänen.pdf (accessed on 23 August 2018).
[69] Lindstad, H., R. Bright and A. Strømman (2016), “Economic savings linked to future Arctic shipping trade are at odds with climate change mitigation”, Transport Policy, Vol. 45, pp. 24-30, http://dx.doi.org/10.1016/J.TRANPOL.2015.09.002.
[85] Macedo, M. et al. (2012), “Decoupling of deforestation and soy production in the southern Amazon during the late 2000s.”, Proceedings of the National Academy of Sciences of the United States of America, Vol. 109/4, pp. 1341-6, http://dx.doi.org/10.1073/pnas.1111374109.
[38] Mangiaracina, R. et al. (2015), “A review of the environmental implications of B2C e-commerce: a logistics perspective”, International Journal of Physical Distribution & Logistics Management, Vol. 45/6, pp. 565-591, http://dx.doi.org/10.1108/IJPDLM-06-2014-0133.
[59] Marine Insight (2018), 9,737 Million Tons Of Goods Shipped On Northern Sea Route In 2017, https://www.marineinsight.com/shipping-news/9737-million-tons-of-goods-shipped-on-northern-sea-route-in-2017/ (accessed on 22 January 2019).
[54] McKinnon, A. (2018), “3D printing: a decarbonising silver bullet”, Manufacturing & Operations Management: Special Feature, http://www.alanmckinnon.co.uk/uploaded/PDFs/Papers/FOCUS%20article%203DP%20-%20SilverBullet.pdf.
[7] McKinnon, A. (2018), Decarbonizing logistics: Distributing goods in a low-carbon world, Kogan Page, London.
[10] McKinnon, A. (2016), “Freight Transport Deceleration: Its Possible Contribution to the Decarbonisation of Logistics”, Transport Reviews, Vol. 36/4, pp. 418-436, http://dx.doi.org/10.1080/01441647.2015.1137992.
[110] McKinnon, A. (2014), “Improving the Sustainability of Road Freight Transport by Relaxing Truck Size and Weight Restrictions”, in Sustainable Practices: Concepts, Methodologies, Tools, and Applications, http://dx.doi.org/10.4018/978-1-4666-4852-4.ch071.
[48] McKinnon, A. (2011), “Improving the Sustainability of Road Freight Transport by Relaxing Truck Size and Weight Restrictions”, Supply Chain Innovation for Competing in Highly Dynamic Markets, Vol. 1974, pp. 185-198, http://dx.doi.org/10.4018/978-1-60960-585-8.ch012.
[51] Mckinnon, A. (2016), “The Possible Impact of 3D Printing and Drones on Last-Mile Logistics: An Exploratory Study”, Built Environment, Vol. 42/4, pp. 617-629, http://dx.doi.org/10.2148/benv.42.4.617.
[65] Melia, N., K. Haines and E. Hawkins (2016), “Sea ice decline and 21st century trans-Arctic shipping routes”, Geophysical Research Letters, Vol. 43/18, pp. 9720-9728, http://dx.doi.org/10.1002/2016GL069315.
[70] Merk, O. (2016), Belt and Road Networks: Some observations, In Along the Silk Roads Conference. Venice, Italy.
[49] MGI (2012), Manufacturing the future: The next era of global growth and innovation, Mckinsey Global Institute, https://www.mckinsey.com/~/media/McKinsey/Business%20Functions/Operations/Our%20Insights/The%20future%20of%20manufacturing/MGI_%20Manufacturing_Full%20report_Nov%202012.ashx (accessed on 23 April 2019).
[35] Mokhtarian, P. (2009), “If telecommunication is such a good substitute for travel, why does congestion continue to get worse?”, Transportation Letters, Vol. 1/1, pp. 1-17, http://dx.doi.org/10.3328/TL.2009.01.01.1-17.
[26] Mokhtarian, P. (2004), “A conceptual analysis of the transportation impacts of B2C e-commerce”, Transportation, Vol. 31/3, pp. 257-284, http://dx.doi.org/10.1023/B:PORT.0000025428.64128.d3.
[14] Montreuil, B. (2011), Towards a Physical Internet: Meeting the Global Logistics Sustainability Grand Challenge, https://link.springer.com/article/10.1007/s12159-011-0045-x (accessed on 31 January 2019).
[92] Moultak, M., N. Lutsey and D. Hall (2017), Transitioning to Zero-Emission Heavy-Duty Freight Vehicules Acknowledgements, ICCT, Washington DC, https://www.theicct.org/sites/default/files/publications/Zero-emission-freight-trucks_ICCT-white-paper_26092017_vF.pdf (accessed on 20 September 2018).
[86] Nepstad, D. et al. (2014), “Slowing Amazon deforestation through public policy and interventions in beef and soy supply chains”, Science, Vol. 344/6188, pp. 1118-1123, http://dx.doi.org/10.1126/science.1248525.
[75] Notz, D. and J. Stroeve (2016), “Observed Arctic sea-ice loss directly follows anthropogenic CO2 emission.”, Science (New York, N.Y.), Vol. 354/6313, pp. 747-750, http://dx.doi.org/10.1126/science.aag2345.
[52] OECD (2017), The Next Production Revolution: Implications for Governments and Business, OECD Publishing, Paris, https://dx.doi.org/10.1787/9789264271036-en.
[106] OECD (2011), Moving Freight with Better Trucks: Improving Safety, Productivity and Sustainability, ITF Research Reports, OECD Publishing, Paris, https://dx.doi.org/10.1787/9789282102961-en.
[18] OECD (2011), OECD guide to measuring the information society 2011., OECD, https://doi.org/10.1787/9789264113541-en (accessed on 24 January 2019).
[22] OECD (2003), Delivering the Goods: 21st Century challenges to Urban Goods Transport., https://doi.org/10.1787/9789264102828-en (accessed on 31 January 2019).
[21] OECD/ECMT (2001), The impacts of e-commerce on transport, in OECD/ECMT Joint seminar 5-6 June.
[5] OECD, WTO and UNCTAD (2018), Reports on G20 Trade and Investment Measures, OECD-WTO-UNCTAD, http://www.oecd.org/daf/inv/investment-policy/19th-Report-on-G20-Trade-and-Investment-Measures.pdf (accessed on 24 August 2018).
[64] Ørts Hansen, C. et al. (2016), Arctic Shipping - Commercial Opportunities and Challenges, https://services-webdav.cbs.dk/doc/CBS.dk/Arctic Shipping - Commercial Opportunities and Challenges.pdf (accessed on 22 January 2019).
[112] Pålsson, H. et al. (2017), “Longer and heavier road freight vehicles in Sweden”, International Journal of Physical Distribution & Logistics Management, Vol. 47/7, pp. 603-622, http://dx.doi.org/10.1108/IJPDLM-02-2017-0118.
[71] Rail Freight (2017), ’Freight tariffs on the New Silk Road must be safeguarded’, https://www.railfreight.com/specials/2017/09/19/freight-tariffs-on-the-new-silk-road-must-be-safeguarded/?gdpr=accept (accessed on 22 January 2019).
[89] Rothkopf, G. (2008), “IV BRAZIL”, in A Blueprint for Green Energy in the Americas, Inter-American Development, http://idbdocs.iadb.org/wsdocs/getdocument.aspx?docnum=945774 (accessed on 8 November 2018).
[11] Route Monkey and WBCSD (2016), Demonstrating the GHG reduction potential of asset sharing, asset optimization and other measures, WBCSD, https://docs.wbcsd.org/2016/11/RoadFreightLab_Report.pdf (accessed on 12 October 2018).
[13] Sánchez-Díaz, I., P. Georén and M. Brolinson (2017), “Shifting urban freight deliveries to the off-peak hours: a review of theory and practice”, Transport Reviews, Vol. 37/4, pp. 521-543, http://dx.doi.org/10.1080/01441647.2016.1254691.
[95] Scania (2016), World’s first electric road opens in Sweden, https://www.scania.com/group/en/worlds-first-electric-road-opens-in-sweden/ (accessed on 21 January 2019).
[90] Schroeder, J. (2010), EPA Deems Sugarcane Ethanol an Advanced Biofuel, Energy.agwire.com, http://energy.agwired.com/2010/02/04/epa-deems-sugarcane-ethanol-an-advanced-biofuel/ (accessed on 8 November 2018).
[96] Schulte, J. and H. Ny (2018), “Electric road systems: Strategic stepping stone on the way towards sustainable freight transport?”, Sustainability (Switzerland), Vol. 10/4, http://dx.doi.org/10.3390/su10041148.
[25] Sener, I. and P. Reeder (2012), “An Examination of Behavioral Linkages across ICT Choice Dimensions: Copula Modeling of Telecommuting and Teleshopping Choice Behavior”, Environment and Planning A: Economy and Space, Vol. 44/6, pp. 1459-1478, http://dx.doi.org/10.1068/a44436.
[103] Serena, R. (2016), Los Megacamiones, una realidad en España | Logística, MUGIE 2015-2016, grupo A, https://logisticaicex.wordpress.com/2016/02/05/los-megacamiones-una-realidad-en-espana/ (accessed on 4 February 2019).
[80] Siemens (2017), eHighway: Innovative electric road freight transport, https://assets.new.siemens.com/siemens/assets/public.1500537078.7bcd4aee10a34603eea30c4e8b8941478c164092.ehighway-2017.pdf (accessed on 21 January 2019).
[58] Smith, L. and S. Stephenson (2013), “New Trans-Arctic shipping routes navigable by midcentury”, Proceedings of the National Academy of Sciences, Vol. 110/13, pp. E1191-E1195, http://dx.doi.org/10.1073/pnas.1214212110.
[17] Tavasszy, L. and J. Meijeren (2011), Modal Shift Target for Freight Transport Above 300 km: An Assessment, ACEA, https://www.acea.be/uploads/publications/SAG_17.pdf (accessed on 12 October 2018).
[76] The State Council Information Office of the People’s Republic of China (2018), China’s Arctic Policy, Belt and Road Portal, http://english.gov.cn/archive/white_paper/2018/01/26/content_281476026660336.htm.
[12] Transport & Mobility Leuven (2017), Commercial Vehicle of the Future A roadmap towards fully sustainable truck operations, IRU, https://www.iru.org/sites/default/files/2017-07/iru-report-commercial-vehicle-of-the-future-en%20V2.pdf (accessed on 12 October 2018).
[56] UIC (2017), Eurasian rail corridors: What opportunities for freight stakeholders?, https://uic.org/IMG/pdf/corridors_exe_sum2017_web.pdf (accessed on 22 January 2019).
[43] UNCTAD (2018), UNCTAD E-Commerce Week 2018 Summary Report, https://unctad.org/meetings/en/SessionalDocuments/dtl_eWeek2018_summary_en.pdf (accessed on 4 February 2019).
[20] UNCTAD (2015), Information Economy Report 2015, https://unctad.org/en/PublicationsLibrary/ier2015_en.pdf (accessed on 31 January 2019).
[97] US DOE (2017), Fuel Cell Technologies Office Multi-Year Research, Development, and Demonstration Plan, http://energy.gov/sites/prod/files/2015/10/f27/fcto_2014_market_report.pdf (accessed on 21 January 2019).
[60] USCG (2017), 2017 Addendum to the United States Coast Guard High Latitude Region Mission Analysis, The Center for Arctic Study and Policy.
[87] Valin, H. et al. (2015), “The land use change impact of biofuels consumed in the EU: Quantification of area and greenhouse gas impacts”, http://pure.iiasa.ac.at/id/eprint/12310/ (accessed on 7 November 2018).
[36] van Loon, P. et al. (2014), “The growth of online retailing: a review of its carbon impacts”, Carbon Management, Vol. 5/3, pp. 285-292, http://dx.doi.org/10.1080/17583004.2014.982395.
[108] Vierth, I. (2017), Research on Modal Split in Sweden, In Workshop on Road Freight Efficiency versus Freight Modal Split. Cambridge, England.
[105] Vierth, I. et al. (2008), The effects of long and heavy trucks on the transport system Report on a government assignment, https://www.diva-portal.org/smash/get/diva2:675341/FULLTEXT02.pdf (accessed on 5 February 2019).
[83] Viktoria Swedish ICT (2013), Slide-in Electric Road System, http://www.viktoria.se, (accessed on 21 January 2019).
[24] Wang, X. and Y. Zhou (2015), “Deliveries to residential units: A rising form of freight transportation in the U.S.”, Transportation Research Part C: Emerging Technologies, Vol. 58, pp. 46-55, http://dx.doi.org/10.1016/J.TRC.2015.07.004.
[42] Wang, Z. and H. Lo (2007), “Urban Development with Sustainable Rail Transit Services”, Transportation Systems: Engineering & Management, Bin72<br/>Times Cited:0<br/>Cited References Count:7, pp. 477-486 756, http://repository.ust.hk/ir/Record/1783.1-39580.
[32] WEF (2017), Shaping the Future of Retail for Consumer Industries, http://www3.weforum.org/docs/IP/2016/CO/WEF_AM17_FutureofRetailInsightReport.pdf (accessed on 1 February 2019).
[55] Westerweel, B., R. Basten and J. Fransoo (2018), 3D printing will impact global trade, but much less than previously thought, https://www.linkedin.com/pulse/3d-printing-impact-global-trade-much-less-than-thought-jan-fransoo (accessed on 4 February 2019).
[50] Wohlers Associates (2018), Wohlers Report 2018 Shows Dramatic Rise in Metal Additive Manufacturing and Overall Industry Growth of 21%, http://wohlersassociates.com/press74.html (accessed on 4 February 2019).
[111] Woodroffe, J. (2017), ‘Assessing high capacity vehicles’, In ITF 2017 Summit. High Capacity Transport: Technologies for tailored network access. Leipzig, Germany., https://2017.itf-oecd.org/high-capacity-transport.
[4] WTO (2018), World Trade Statistical Review 2018, http://www.wto.org/statistics.
[68] Yumashev, D. et al. (2017), “Towards a balanced view of Arctic shipping: estimating economic impacts of emissions from increased traffic on the Northern Sea Route”, Climatic Change, Vol. 143/1-2, pp. 143-155, http://dx.doi.org/10.1007/s10584-017-1980-6.
[39] Zanni, A. and A. Bristow (2010), “Emissions of CO2 from road freight transport in London: Trends and policies for long run reductions”, Energy Policy, Vol. 38/4, pp. 1774-1786, http://dx.doi.org/10.1016/J.ENPOL.2009.11.053.
Notes
← 1. Many B2B transactions do not necessarily impact transport, either because they do not change previous transport patterns in a significant way or, as is increasingly the case, because they do not involve the transportation of any goods at all (e.g. web development or other digital services).
← 2. The substitutability or complementarity between online and in-store purchasing may also vary according to product type and frequency of purchase. However, while consumption-related e-commerce has an uncertain net impact on travel, service-related e-commerce is likely to decrease travel (European Commission, 2001).
← 3. The Kra Canal would supplement current flows through the Strait of Malacca, the world’s busiest maritime corridor. However, plans for its construction have never materialised over the course of the past century, due to financial costs and environmental concerns. Recently, China and Thailand have explored the idea at conferences in 2017 and 2018.