This chapter seeks to illustrate the importance of technological development in carbon management. It is worth noting that while most technologies have some relation to climate action, the perspective presented here is much broader and consistent with the message of avoiding ‘sustainability tunnel vision’. It will be apparent that there is very large scope for ‘hybrid’ technologies, involving more than one type of technology, such as a combination of bio- and nano-technologies.
Carbon Management: Bioeconomy and Beyond
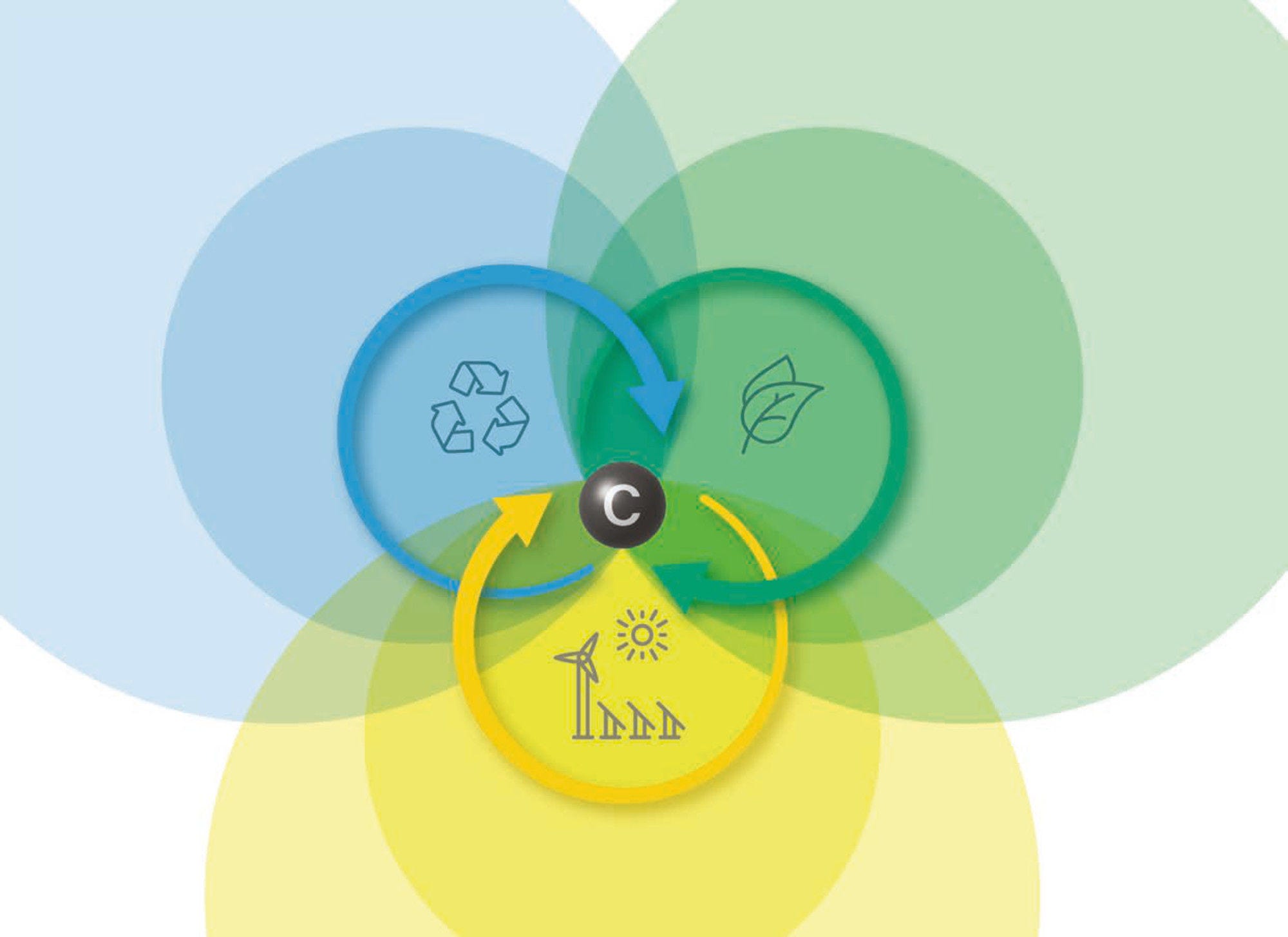
4. Carbon management technologies
Abstract
While classic biological production is indispensable for most food and feed, fibres and other complex molecules like performance chemicals and biopharmaceuticals, the chemicals industry could concentrate more on CO2 as a feedstock (Carus et al., 2020), despite the thermodynamic difficulties of doing so, using hydrogen as a supplementary reagent and energy carrier. The synthesis of simple organic chemicals and fuels are amenable to new processes based on biotechnological (e.g., gas fermentation), new strategies for chemical synthesis and nanotechnology. All of this would relieve pressure on land and increase circularity, major goals for sustainability.
In the following sections, various technologies are described through their potential application and impact, rather than the technologies per se. Table 4.1 attempts to categorise some of the technologies described, both by the energy/land use system they affect and the SDG(s) that they address. As alluded to above, governments tend to pick out wanted consequences, while governments must also be alert to the unwanted.
In this description, revisiting the key value chains of bioproduction (i.e., the bioeconomy) is the start-point, followed by the new alternatives to carbon-based products, such as recycling of carbon and direct air capture of CO2. This also includes a description of technologies involved in hydrogen production, as hydrogen is increasingly an essential reagent in carbon-based value chains. Finally, the development of carbon capture and sequestration is outlined, being perceived to be essential for managing overshoot and unavoidable emissions.
Table 4.1. Technologies described and their relation to energy/land use system and SDGs
Technology |
Energy/Land use system |
SDGs |
||
---|---|---|---|---|
|
Primary |
Other |
Primary |
Other |
N-fixing cereal crops |
Agriculture |
Waste |
2 |
3, 6, 10, 13, 14, 15 |
Ammonia |
Agriculture |
Industry, Transport |
2 |
7, 9, 12, 13, 15, |
Soil |
Agriculture |
Forestry, Waste |
2 |
3, 10, 12, 13, 15 |
Chemical recycling |
Waste |
Industry |
12 |
6, 9, 11, 13 |
Anaerobic digestion |
Agriculture |
Power, Waste |
7 |
11, 13, 15 |
Methanol |
Industry |
Transport |
12 |
8, 7, 8, 9, 13 |
DAC |
Power |
Industry |
13 |
8, 9, 12, |
Wood in construction |
Forestry |
Buildings |
11 |
9, 12, 13, 15 |
Cement/concrete |
Buildings |
Waste, Mining |
11 |
12, 13, 15 |
BECCS/DACCS |
Power |
Industry, Transport, Forestry, Waste |
7 |
8, 9, 12, 13 |
Nanotechnology |
Industry |
Power, Transport, Buildings, Agriculture, Waste |
12 |
3, 4, 6, 7, 8, 9, 11, 12, 13, 15 |
Synthetic biology |
Industry |
Transport, Agriculture, Waste |
12 |
2, 3, 4, 6, 7, 8, 9, 11, 13, 14, 15 |
Synthetic chemistry |
Industry |
Power, Transport, Buildings, Agriculture, Waste |
12 |
3, 4, 6,7, 8, 9, 11, 13, 14, 15 |
Note: Energy/land use systems: power, industry, transport, buildings, agriculture, forestry, and waste.
SDGs goals: 1: No Poverty, 2: Zero Hunger, 3: Good Health and Well-being, 4: Quality Education, 5: Gender Equality, 6: Clean Water and Sanitation, 7: Affordable and Clean Energy, 8: Decent Work and Economic Growth, 9: Industry, Innovation and Infrastructure, 10: Reduced Inequality, 11: Sustainable Cities and Communities, 12: Responsible Consumption and Production, 13: Climate Action, 14: Life Below Water, 15: Life on Land, 16: Peace and Justice Strong Institutions, 17: Partnerships to achieve the Goal.
BECCS and DACSS differ in that BECCS involves providing clean energy, while DACCS is purely storage and requires clean energy.
A fresh look at bioproduction
A fresh look at bioproduction is meant to reflect the realisation that biomass and bio-based production cannot replace all fossil-based production in a manner that would preserve other sustainability necessities, such as biodiversity.
Projections suggest that more than 840 million people worldwide will be undernourished by 2030. By century end, some 10 billion people will need carbon-neutral food systems that are resilient to extreme weather and resistant to current and emerging pathogens while respecting planetary boundaries (Fraser and Campbell, 2019).
In fact, some of the most significant challenges in climate change relate to agriculture. Agriculture, forestry and other land use (AFOLU) activities accounts for around 23% of total net anthropogenic emissions of GHGs (IPCC, 2020). Environmental damage caused by the food system could increase by 50-90% by 2050 if technological change and dedicated mitigation measures are not deployed (Springmann et al., 2018).
Attempts to improve crop yields by conventional plant breeding over the last 50 years or so have been characterised by an improvement of about 1% per year (Foyer et al., 2017), while what is probably needed as a year-on-year improvement to 2050 is 1.7%. One promising way to achieve such a step increase is to improve the efficiency of photosynthesis (Kromdijk et al., 2017).
Photosynthesis is the primary determinant of crop yield (Simkin et al., 2019), but the maximum overall photosynthetic efficiency of plants is only 3-6% of total solar radiation and in practice considerably lower. Given the relatively slow progress in crop yield improvements, de Souza et al. (2022) described a remarkable improvement in a bioengineered strain of soybean. In replicated field trials, they increased photosynthetic efficiency and seed yield by up to 33% without a decrease in protein or oil content.
Biotechnologies coming of age
In the landmark OECD bioeconomy publication (OECD, 2009), biotechnology was envisioned to be the backbone of the emerging bioeconomy. While developments in biosciences and capabilities in bioengineering have been tremendous, biotechnology is still far from reaching its full potential as an enabler of sustainable bio-based production. However, many examples of an increasing impact are visible.
Breeding for climate resilience. One important area is genomic selection of crops with enhanced ability to grow under multiple climate-related stress conditions (Budhlakoti et al., 2022). This could be especially important in the future regarding resilience to drought and high temperatures. For example, recently CRISPR tools have been effectively applied to elucidate drought and saline environments tolerance mechanisms in plants (Shelake et al., 2022).
Pest-resistant genetically engineered crops (Tabashnik and Carrière, 2017). Some plant pathogens have already expanded their host range or distribution, at least partly as a result of climate change (Gullino et al., 2022). It is considered that genetically engineered crops can be critical components of an integrated pest management (IPM) plan in both developed and developing countries (Anderson et al., 2019).
Increased nutritional value. Paine et al. (2005) described a strategy to increase the nutritional value of golden rice through increasing the pro-vitamin A content. Other target crops for biofortification are among the most important globally, especially corn and wheat (Hefferon, 2015).
The emergence of novel biomaterials based on biotechnology. Instead of trying to just produce ‘drop in’ bio-based equivalents, completely new bio-based materials with superior engineering characteristics are possible. For example, biotechnology-based spider silk has applications in microphones in hearing aids and cell phones, aircraft structural applications, even in high-value cosmetics (Bell et al., 2021).
More versatile microbial production. Traditional fermentations based on sugars or gases are moving from solely ethanol (as a fuel) to a wider range of chemical building blocks and higher value-added products (e.g., Liew et al., 2022). Gas fermentation is the perfect example for this report as using CO2 rich industrial waste gases, including those originating from bioresources, relieves pressure on crops and thus land.
Engineering biology, the missing link in biomanufacturing
Synthetic biology may be described as the application of science, technology and engineering to facilitate and accelerate the design, manufacture of genes leading to modification of genetic materials in living organisms. It aims to bring an engineering approach to biotechnology by design and engineering of biologically-based parts, novel devices and systems, as well as redesigning existing, natural biological systems. It might be regarded as a technical evolution of metabolic engineering, a technology from the 1990s, which can be described as the use of genetic engineering to modify the metabolism of an organism. It is fairly recently, however, that engineering/synthetic biology has been explicitly linked to climate change e.g., DeLisi (2019) and sustainable development (French, 2019).
Complexity of life forms and their cellular machinery has forced life scientists into a reductionist method of experimentation by examining one factor at a time (OFAT). By contrast in modern manufacturing R&D, design of experiments (DOE) is the systematic method of investigating the relationship between multiple factors and their impact on the process simultaneously1. This is very difficult, if at all possible, to do manually in biology. However, the biofoundry concept is allowing this method in bioengineering.
Biofoundries are highly automated facilities that comprise the extensive and coordinated use of laboratory robots (Figure 4.1a) that are programmed to perform specific tasks according to a workflow (Kitney et al., 2019). A hallmark of modern manufacturing is the separation of design from manufacturing. Here, the biofoundry is the design hub, and it can be geographically separated from a biomanufacturing plant by any distance as the design can be transferred digitally. The combination of biodesign tools (BioCAD), including handling of “big data” and multivariate analysis, and biofoundries is rapidly producing a new type of biology - digital biology - that could revolutionise bio-based production by being the important design link to a number of different types of bioproduction and sectors (Figure 4.1b).
Figure 4.1. The biofoundry as the missing link in biomanufacturing

Note: BRC – Biological Resource Centre; DBTL – the Design-Build-Test-Learn cycle.
Source: Figure 8(a) – Courtesy of the Edinburgh Genome Foundry, University of Edinburgh
Technologies for smart and precision agriculture
Agriculture is a sector ripe for technological change that could improve its sustainability. A few examples are given here, with an emphasis on technologies that reduce the demand for critical resources such as land, water and fertilizers. Several of these technologies have been scrutinised for their ability to reduce emissions and complement conservation agriculture (Northrup et al., 2021).
Plant-based alternatives to meat and dairy. These may have the greatest sustainability impacts in food and agriculture. A recent report2 suggested that improving and scaling up the production of meat and dairy alternatives results in three times greater GHG reductions compared with investment in green cement technology, seven times more than green buildings and eleven times more than zero-emission cars.
Vertical farming. Growing crops under controlled indoor conditions saves water, labour and fertilizer, nullifies runoff, and can drastically shorten the cold chain3, all the way down to local production in vertical city farms. Vertical farming is currently expanding rapidly, with many investors, start-ups and established greenhouse industry companies entering the space to satisfy the consumer demand for fresh and local food (Van Gerrewey et al., 2022). As with many of these potentially disruptive technologies, there are concerns over the requirement for energy, which accounts in large part for higher costs for these crops, partly responsible for several unsuccessful initiatives (Beh and Dent, 2022).
Smart tractors and machinery. Ninety percent of all energy invested in cultivation is to repair damage to crops and soil caused by tractors4. GPS-guided tractors ensure that all traffic follows the same routes, maximising fuel economy and minimising crop damage. But the range of functions is much larger than simply driving. For example, path-generating algorithms can calculate, among others, the size of implements, coverage area patterns, and the implement turn radius (Tripathi et al., 2022).
Precision farming. Robotics and the use of advanced sensor technology are being introduced to optimise resource efficiency. In precision farming the field is divided into a virtual grid where water and fertilizers can be administrated according to varying needs. Precise administration of pesticides allows for reduced environmental burden.
Tackling food waste and food distribution challenges. Using food waste as a raw ingredient demonstrates cascading use and circularity. Instead of throwing away 44% of all bread baked it can be used again by, for example, fermenting it to beer, a technology already in existence through companies such as Toast Ale5. The CO2 resulting from the fermentation is food-grade and is easily collected for use in carbonated drinks.
Enzymatic upgrading of bio-residuals. Enzymes obtained from microbes are commonly known as the key factor in modern detergents. However, enzymes are also important in improved utilisation of biomaterials and food. In Norway, Borregaard6 is developing enzymes to extract fermentable sugars from solid wood, while several companies are enzymatically converting off-cuts and residuals from the marine industry7 or poultry8 into high end products in food and feed.
The use of insect bioreactors is an example of a new type of bioproduction. Cultivating the larvae of various insect species can very efficiently convert carbohydrate-rich residuals into protein and fat, illustrating upcycling of biowaste from e.g., the food industry.
Anaerobic digestion. When opportunities for functional utilisation have been exhausted, anaerobic bacterial culture can process biowaste into methane for heat and electricity, even at individual farm scale (IEA Bioenergy, 2015; see Recycling of wet organic material.
The enigma of meat
As the global middle class increases in size, the demand for meat in the diet increases. To date, livestock occupies almost 80% of global agricultural land, yet produces less than 20% of the calories supply (Poore and Nemecek, 2018). Beef and mutton are the main culprits; the land area they require is up to 100 times larger than for cereals9. To keep up with food demand, 13 billion hectares of forest area are lost each year to agricultural uses. This has detrimental effects on regional water availability, soil fertility, biodiversity and climate change.
While biotechnology-based and plant-based meat substitutes are slowly gaining popularity10 and there is tentative evidence of significant improvements in sustainability compared to conventional meat11, science advances will need to be accompanied by behavioural changes, and policy can play important roles. In the large (1.2 million people) UNDP climate policy survey published in 2021, one of the two least popular policies overall was plant-based diets (UNDP, 2021).
Breeding for efficiencies in the production of meat and milk, e.g., selection of traits for higher protein content, is increasingly guided by modern methods like genomic markers or CRISPR (e.g., Singh and Ali, 2021). Genomic selection is now a de facto standard in leading dairy cattle breeding programmes (Obšteter et al., 2021). The same development is seen in poultry, where genomic selection is used to improve feed conversion ratio and leg strength in chicken. Aviagen (Scotland) became the first company in 2012 to include genomic information in its poultry R&D breeding programme.
The emissions from agriculture are not simply CO2 as, in fact, methane and nitrous oxide related to animal husbandry constitute the dominant direct emissions, both of which are much more potent greenhouse gases than CO2. This is one factor that complicates the accounting of agricultural emissions. More research is needed to elucidate how emissions of methane and nitrous oxide contribute to temperature change and how it can be mitigated.
One strategy to reduce methane emissions would be genomic selection of cattle with lower methane production in their gut (Lassen and Difford, 2020). Another strategy which is currently explored, is adding chemical compounds to the feed that selectively knocks out the methanogenic bacteria present in the gut flora. One such compound is the commercially available 3-nitrooxypropanol. A naturally occurring alternative would be bromoform, which can be administrated by adding a small amount of certain red seaweeds to the feed (Kinley at al., 2020). This is spurring the search for additives from a wider range of seaweeds (Mihaila et al., 2022). The Australian company Rumin8 has ongoing trials with an additive that consistently demonstrates more than 85% methane reduction, which equates to two tonnes of carbon emissions removed from the air, per cow, per year12.
Fertilizer and its alternatives
Ammonia (NH3) is the key nitrogen source in making mineral fertilizer. It contains no carbon, and yet its production process is responsible for about 1.8% of global CO2 emissions (Figure 4.2), making it the largest CO2-emitting chemical industry process (IEA, ICCA, DECHEMA, 2013). The process consumes some 1.8% of global energy output each year (Royal Society Policy Briefing, 2020) and around 3-5% of global natural gas (Licht et al., 2014). Fortunately, technological alternatives exist, and major producers of fertilizers are currently exploring production of ammonia where natural gas is replaced with hydrogen produced by electrolysis of water using renewable power.
Meanwhile, Australian researchers have discovered an electrochemical method for production of ammonia from air that relies on a phosphonium cation working as catalyst at ambient temperature and pressure (Jasi, 2022). In contrast to the conventional Haber-Bosch process, this can be miniaturised to container-sized production units generating 1 tonne per day. Such units could be located and used on farms, thus allowing farmers to make fertilizer on demand, an approach consistent with a small-scale distributed manufacturing paradigm.
It should also be noted, however, that there are large environmental problems created by the overuse of mineral fertilizers13, especially the eutrophication of water courses (Brownlie et al., 2022). Within the IPCC accounting framework, emissions from the manufacture of fertilizer manufacture come under ‘energy’ and effects related to its use, e.g., eutrophication, are not considered in this framework.
Nitrogen fixation for cereal crops would lower the need for mineral fertilizers, or in the best case nullify their requirement. There are two basic technologies. The first is to use nitrogen-fixing engineered bacteria to colonise roots of cereal crops – here there is one commercialised strain that has completed trials and safety procedures (Wen et al., 2021). The second, which is still developing, aims to create novel crops that fix their own nitrogen from the atmosphere (e.g., Rosenblueth et al., 2018).
Figure 4.2. The top GHG emissions producing chemicals

Note: Ammonia, containing no carbon itself, is far above the other highest organic chemicals in emissions. Of all chemicals, its production also has the highest energy demand. The figures are for 2010.
Source: Reproduced from Royal Society Policy Briefing (2020).
Soil, the forgotten resource
Long overlooked by policy makers, soil is the ultimate resource in the bioeconomy: more than 95% of all food is derived from cropland soil and its microbes (Gore, 2013). By 2050, the world will need to produce 50-70% more food, increasingly under drought conditions (Cook et al., 2015), increased fire frequency14, and on degraded soils (Karlen and Rice, 2015). Meanwhile permafrost thaw could have disastrous consequences by releasing vast quantities of CO2 and methane to the atmosphere (Schuur et al., 2015).
As important as it is, the ecosystem services provided by soils are grossly undervalued (Baveye et al., 2016). Soil accounts for some 20% of natural removal of human CO2 emissions (European Commission, 2007), but it is very easily damaged, and very slow to renew. It is estimated that in the past two centuries, soil organic carbon has decreased by 8% globally. Some 20% of the surface of the European Union is subject to soil erosion at a rate of 10 tonnes per hectare per year, leading to a loss of productive land of 1 000 km2 every year. It is hardly surprising, then, that the FAO regards soil as a finite, non-renewable resource15. It is currently being depleted at a faster rate than it is being formed (Handelsman, 2021).
Soil microbiomes
The true value of soil becomes obvious when seen as a biological resource, rather than a simple geochemical entity. Soil microorganisms fix nitrogen, enabling fertilisation. As soil microorganisms are largely responsible for cycling of soil organic carbon (SOC) and other nutrients, their irreplaceable and essential role in climate feedback is now recognised (Jansson and Hofmockel, 2020). If soil has been forgotten as the ultimate resource, it is because the role of soil microbiota in forming and sustaining soils has historically been overlooked (Coban et al., 2022). A greater association between soil microbiomes and the UN SDGs (D’Hondt et al., 2021) would bring greater political attention to soils.
Technologies such as environmental genomics and metabolomics are now untangling the multiple roles that soil plays by detailed examination of the complex web of life that soil hosts – now known as the soil microbiome, comprising bacteria, archaea, viruses, fungi and protozoa. However, there is still a lack of information with respect to the major drivers that trigger microbial community composition and function on all scales (Nannipieri et al., 2019). To this end, the Earth Microbiome project16 has provided a number of important but voluntary standards for the analysis of soil microbiomes. These facilitate the comparison of data from single projects with studies by other researchers in a meta-analysis.
Carbon farming
Carbon farming constitutes agricultural techniques aiming to reduce GHG emissions by increasing the content of carbon in soil, reaching beyond but also including traditional recycling of biomaterials through manure and leaving straw and crop residuals on the field. While these are management practises rather than technologies (see biochar discussed below), they improve soil health and sequester carbon in combination with important co-benefits, including increased soil water-holding capacity, bioavailability of nutrients, biodiversity, and resilience (Zomer et al., 2017).
The EU Farm-to-Fork strategy17 intends to reward farmers and foresters implementing carbon farming practices. However, an obstacle to making a reward system is the realistic quantification of the impacts of these practices, a dilemma recognised in the United States SMARTFARM case study (see Recycled and atmospheric carbon). The conservation practices include18:
afforestation and reforestation that respect ecological principles favourable to biodiversity and enhanced sustainable forest management
‘agroforestry’ and other forms of mixed farming combining woody vegetation (trees or shrubs) with crop and/or animal production systems on the same land
use of crops and conservation tillage that protect soils, reducing soil loss by erosion and enhancing soil organic carbon on degraded arable land
targeted conversion of cropland to fallow or of set-aside areas to permanent grassland
restoration of peatlands and wetlands that reduces oxidation of the existing carbon stock and increases the potential for carbon sequestration.
Biochar and CCS
The International Biochar Initiative19 defines biochar as “solid material obtained from the thermochemical conversion of biomass in an oxygen-limited environment”. As for carbon farming discussed above, several relevant benefits are promoted for biochar, among them improvements to soil biodiversity and soil fertility. The carbon in biochar is purported to be stable for perhaps thousands of years, raising the concept of pyrogenic carbon capture and storage (PyCCS) (Werner et al., 2018). Schmidt et al. (2019) demonstrated that PyCCS can achieve carbon sequestration efficiencies of greater than 70%, a level considered to be an important threshold to allow PyCCS to become a relevant negative emission technology.
Compostable plastics and soil amendments
Compostability of plastics becomes essential for all applications in which the materials used are highly likely to be polluted by food residues, such as organic waste bags, food packaging, and coffee capsules. When contaminated with food waste, recycling of plastics is not feasible (Schyns and Shaver, 2020), neither with methods designed for plastics, nor as biowaste; thus if these plastics are not biodegradable the probability is high that such waste materials end up in landfills (OECD, 2022).
Two of the case studies for this report, one from Germany (see chapter 5) and one from Italy (see chapter 5) describe the development of a biodegradable and compostable plastic value chain (products such as bags, packaging, or cutlery) together with an efficient system for biowaste collection and recycling. The objective is to make the mixed waste suitable for organic recycling and for the creation of high-quality compost.
Recycled and atmospheric carbon
Recycling of wet organic material
Wet organic wastes such as sewage sludge and agricultural slurries require to be dried before their use in thermochemical processes. That drying process is very energy intensive. The anaerobic digestion of these wet wastes obviates the need for drying, and results in a useful fuel (biogas) which can also be used as a feedstock.
Anaerobic digestion of sewage sludge to produce biogas (a mixture comprising mainly methane and CO2), has been used for over a century in the biological treatment of wastewater. It stabilises sewage sludge by removing pathogens and at the same time captures a substantial part of the energy in the organic material. The biogas can subsequently be converted to electricity, which can be sufficient to power an entire wastewater treatment plant (Schwarzenbeck et al., 2008), adding to the environmental and economic sustainability of such plants, while decreasing grid dependence.
Anaerobic digestion is highly scalable. It has been perfected down to individual farm level (Figure 4.3), where a variety of waste materials can be converted to biogas (e.g., sludge, grass, solid manure, chicken manure and straw). Moreover, the effluents after anaerobic digestion are better balanced to meet crop needs than raw manure slurries. This reduces the need for supplementary chemical nitrogen and phosphorus fertilizers (Massé et al., 2011), while reducing GHG emissions (Siegmeier et al., 2015).
Figure 4.3. Farm-scale anaerobic digester, Jelsum, Netherlands

Note: The plant is capable of ‘gas to grid injection’, the gas is produced from 7 000 tonnes of manure and 7 000 tonnes of waste products. The plant produces the equivalent of the natural gas for 750 households. The project was realised with a Top Sector Energy subsidy from the Netherlands Ministry of Economic Affairs.
Source: Courtesy of HoSt, www.host.nl/en.
There are many scales and classifications of anaerobic digestion plants, reviewed comprehensively by O’Connor et al. (2021). They posit that small-scale anaerobic digesters (SSADs) offer potential for expansion of the technology in Europe due to the ability to operate economically in small to medium-sized farms with lesser available biomass quantities. National biogas subsidy schemes can strongly support the uptake of SSADs.
Of the European Union countries, Germany in particular has oriented itself towards small-scale biogas plants. German SSAD plant installations increased after the German government introduced an amendment to the Renewable Energy Source Act (Erneuerbare-Energien-Gesetz)20. This amendment provided a special allowance for biogas plants with an installed electrical capacity up to 75 kW, greatly improving the economics of operating such a plant. In a similar policy approach, the Flanders region of Belgium has seen rapid uptake of SSAD plants in recent years, many of the installations having been supported through the Flemish Climate Fund21. The US Federal Energy Regulatory Commission Order 2222 (FERC-222222) incentivises biogas production on United States dairy farms through electricity policy changes (Erickson et al., 2023).
The US EPA offers an Anaerobic Digestion Screening Tool23 to stakeholders such as farmers to assess the feasibility of the technology on a case-by-case basis. Such guidance is important as scale of operation can determine the cost of electricity generation. This is especially true when anaerobic digestion is linked in policy via feed-in tariffs (FITs) (UK Department of Energy and Climate Change, 2016). A FIT is a national policy mechanism that provides payments and long-term contracts to renewable electricity producers, proportional to the amount of power generated.
Treatment and recycling of dry solid waste
The organic fraction of municipal solid waste (MSW) should ideally be biodegradable and compostable, and there are technologies available for both domestic and industrial composting. Indeed, industrial composting has become a widespread organic MSW treatment procedure (Siles-Castellano et al., 2021). More challenging as a solid waste is plastic waste as the vast majority of fossil-based plastics are not biodegradable and can remain in the environment for decades or longer. International policy interventions to reduce plastic waste since 2005 lack robust monitoring and enforcement measures and are failing to contain the plastics in the oceans dilemma.
Mechanical recycling of polymers
Mechanical recycling is already well established, but it is hardly adequate, as the many reports on plastic garbage in the oceans testify (e.g., IUCN, 2021). Eriksen et al. (2023) estimate there are some 170 trillion plastic fragments in the oceans. In the EU recycling hierarchy mechanical recycling is considered second-best to reuse. Here, plastic products would be sorted and melted and/or remoulded into new products. This is hampered, however, by several obstacles related to the product design. Many products are composites containing different materials and products as well as pigments and other additives which are hard to remove.
More advanced technologies for automated sorting and pre-processing combined with regulations incentivising plastic products designed for recycling may improve the opportunity for cascading use. Meanwhile, plastics are typically downcycled to less advanced products like flowerpots and construction materials. While not optimal, its use in bricks, blocks and tiles would mitigate the negative effects of mining (Lamba et al., 2021).
Chemical recycling of polymers and other dry waste
Chemical recycling offers different opportunities by turning plastics or dry mixed waste into a depolymerised secondary raw material (Modesti et al., 2018), thereby closing the carbon loop and creating circularity (cradle-to-cradle) (Figure 4.4). There are at least three mature and industrialised chemical recycling technologies and there are several others at lower stages of development (Solis and Silveira, 2020). They all operate at elevated temperatures, but the intermediate products and thus the following downstream conversion methods and applications will vary.
Pyrolysis, which typically take place at 400-600°C in the absence of oxygen, converts the waste into a gas effluent, an oil fraction and a solid carbon fraction (biochar), which can all be further processed or utilised. The ratio between the oil and the biochar fractions depends on the temperature gradient, as fast pyrolysis typically maximises the oil content. Gasification takes place at higher temperatures, usually close to or above 1 000°C, in the presence of controlled amounts of oxygen. Here, some of the waste feedstock is incinerated to maintain the high temperature (creating some CO2), while most is converted to synthesis gas, a mixture of CO, CO2 and hydrogen, where the ratio depends on the composition of the feedstock.
These methods can be adapted to many types of feedstocks, not only plastics, but a large variety of industrial and municipal solid wastes, the dry organic residual from anaerobic digestion or low grade bioresources. Synthesis gas is a particularly interesting and versatile intermediate, as it can be combined with mixtures of CO2 and hydrogen utilised in CCU or DAC, as will be described below.
To date, there are too few operations at full scale (Figure 4.5) to determine which of these technologies has the greatest economic or environmental advantage. Indeed, this may not be clear-cut in all circumstances as local conditions may dictate a specific technology e.g., volumes of feedstock available, consistency of quality and supply, local policy for recycling, and gate fees24. LCA is the tool of choice at present for assessing the feasibility of the technology, but as in other instances, this may only be a useful start-point.
Figure 4.4. Chemical recycling increases the circularity of plastics

Note: While mechanical recycling is decades old, chemical recycling technology is relatively new.
This should point policy makers in familiar directions e.g., public research funding, R&D subsidy or other forms of public-private partnership as de-risking instruments. In Europe, for example, there are 225 million tonnes of municipal waste generated per year, or about half a tonne per person25 of which only about 30% is recycled, 27% is incinerated and 24% still ends up in landfill. This includes 25.8 million tonnes of plastics waste where only about the same fraction (30%) is being collected for mechanical recycling. (European Commission, 2018). There is clearly a need to increase the market and profitability in recycling, whether by mechanical or chemical processes.
Figure 4.5. Shell is building a chemical recycling plant in Singapore

Note: Slated to start production in 2023, the unit at Shell’s manufacturing site on Pulau Bukom will be the largest in Asia and Shell’s first globally, with a capacity of 50 000 tonnes per year, equivalent to the weight of about 7.8 billion plastic bags.
Source: Courtesy of Photographic Services, Shell International Limited.
Technologies for utilisation of industry flue gases (CCU)
C1 pathways, especially methanol
As shown in Figure 4.6, a large proportion of organic (carbon-containing) chemicals are made from a small number of platform molecules, methanol, three aromatics (xylene, toluene and benzene) and the two olefines (ethylene and propylene). The latter can in principle be synthesised from the C1-molecule methanol, underscoring its importance as a key intermediate. Add butadiene to this list, and these seven molecules constitute the platform that serves more than 90% of organic chemical production, including tens of thousands of products (Tickner et al., 2021). Furthermore, ethylene and propylene are used to make polyethylene and polypropylene, which together make up more than 50% of all non-fibre plastics (Geyer et al., 2017), and are in everyday use in many bulk applications around the world.
Figure 4.6. Summary of routes to the major platform chemicals

Note: Methanol can be processed to five other major platforms.
Source: Galán-Martín et al., 2021
The production of methanol, typically from natural gas, has nearly doubled in the last decade, and demand for methanol is going through a period of rapid expansion (SOTACARBO, 2020). Replacing fossil methanol with a CO2-based route (CCU) could lead to a closed-carbon chemical sector, perhaps even orchestrating a negative emissions balance, sequestering CO2 in long-lived plastics or construction materials (Hepburn et al., 2019).
The main objection to using CO2 as a feedstock, however, is that its thermodynamic stability makes a reaction with hydrogen to methanol very energy demanding, in a typical case provided by hydrogen in the form of energy rich hydrogen gas (H2). However, the CO2 and hydrogen feedstocks can both be generated from either fossil or renewable resources. This flexibility of feedstock together with its general utility, makes CO2-based methanol production an especially interesting case in carbon management.
Methanol can also be produced from biomass and has been made from domestic waste at commercial scale my Enerkem of Canada26. It will typically go via gasification of the bioresource to create synthesis gas (CO, CO2 and H2), followed by essentially the same downstream processes as used in the CCU route. To date, the green routes to this vital platform chemical remain uncompetitive but could be competitive by 2030 given some assumptions (Schorn et al., 2021). What is clear, however, is that the main barrier to renewable methanol uptake is not a lack of technology, but its higher cost compared to fossil fuel-based alternatives, and the potentially limited availability of renewable energy.
Replacing fossil methanol with a renewable alternative could offer a significant reduction in CO2 emissions in the chemical sector. Moreover, being a currently used platform chemical would allow for a simple drop in substitution without the need for major changes in process design and retrofitting of manufacturing infrastructure.
Outside its central importance in the chemicals industry, renewable methanol is being suggested as a solution in another hard-to-abate sector – shipping (Andersson and Salazar, 2015). While light vehicles are increasingly electric, replacement of fossil fuels in heavy transport such as aviation and shipping is a bigger challenge. Mærsk, the shipping giant, recently increased its investments in renewable methanol-fuelled container vessels27.
C2 – C4 pathways
A direct non-fossil production of longer carbon molecules, such as the alcohols; ethanol, propanol, and butanol (C2-C4), is typically done by microbial fermentation. However, the cost, the land, nutrients and energy resources required to produce sugar substrates for the microbes makes this route to simple commodity platform chemicals less attractive (Scown and Keasling, 2022). In order to avoid conflicts with food production, so-called second-generation sugars from e.g., cellulose could be used, but this technology is still immature and, in most cases, prohibitively expensive.
After several decades of work on (ligno)cellulosic waste as a feedstock, the technology is still unproven. New breakthroughs in consolidated bioprocessing (CBP) research have been qualified by the potential needing to be “improved by metabolic and process engineering for higher yields of ethanol production and plant biomass utilization” (Maleki et al., 2021).
Another, perhaps more near-term route to C2-C4 chemicals is gas fermentation. Here, microbes use carbon gases such as CO2 or CO as carbon source and H2 gas as hydrogen and energy source, thus providing a biotechnological approach to utilising the same gases as described above for chemical methanol production. Again, the main benefit is flexibility in the source of such gases and if industry flue gases are being used, there is virtually no requirement for land.
The microbial fermentation of waste industrial gases to useful products such as ethanol (Liew et al., 2016), acetone (Liew et al., 2022) and animal feed (Pander et al., 2020) is becoming reality. These processes can still be improved, however, and genetic engineering and synthetic biology are capable of further developing gas fermentation as a highly versatile manufacturing platform with great potential for scaling.
There are various ways that fermenting waste gases to products contributes to sustainability. It is one of the emerging technologies of CCU (Zhu, 2019), effectively a value-adding strategy compared to CCS. A cradle-to-grave LCA for the production of ethanol by gas fermentation estimated a 67% GHG reduction using steelmaking off-gases compared to conventional fossil gasoline (Handler et al., 2016). Off-gas from steel mills is a convenient feedstock because they comprise a high proportion of carbon monoxide (CO), which in contrast to CO2 contains energy. If renewable hydrogen is added, the carbon efficiency of the fermentation process increases, i.e., the amount of carbon incorporated into the ethanol product.
As already mentioned, gas fermentation can also use synthesis gas produced via gasification (Liakakou et al., 2021) from a range of sources such as agricultural residues, forestry waste, sorted plastics or municipal solid waste, as well as CO2 from any source, including direct air capture (DAC) supplemented with hydrogen28. When the CO2 (or part of it) originates from biogenic material or from DAC, the products made can in principle be fully renewable.
Fossil feedstocks: electrifying the process
Figure 2.2 suggests that fossil raw materials will still be in use in the chemicals industry for decades to come. That being the case, there is a need to abate emissions within existing oil refineries and chemical production plants until such times as fossil replacement technologies are feasible. Steam cracking29 is a crucial process in the chemical production chain and is of one of the most energy-intensive processes in the industry, resulting in a large source of its CO2 emissions. Typically steam cracking is done in furnaces at around 850°C by burning fossil fuels like natural gas. If cracking can be electrified using sustainable solar and wind power instead of fossil-based fuels, CO2 emissions can be greatly reduced.
The German Federal Ministry for Economic Affairs and Climate Action has granted EUR 14.8 million to support the development of a novel furnace technology with BASF, SABIC and Linde at the BASF Verbund site at Ludwigshafen. By using electricity from renewable sources instead of natural gas, the new technology has the potential to reduce CO2 emissions by at least 90% compared to technologies commonly used today30. This is a world first demonstration plant for large-scale electrically heated steam cracker furnaces.
Microbial single cell protein for food and feed
Protein is an essential component of food and feed. Microbial production of protein, so-called single cell protein (SCP), was a full-scale industry 50 years ago but started to fail during the 1970s oil shocks (Groenewald et al., 2014). Today, pressure on natural resources has revived interest in SCP from various sources, e.g., first and second generation sugars. As for chemicals discussed above, gas substrates such as methane, waste-to-syngas and off-gases (Jones et al., 2020) have all become interesting alternatives to sugar-based fermentation. An interesting theoretical study has suggested that gas fermentation based on energy from sun panels could increase protein yield five-fold per area, compared to sugar fermentation and fifteen-fold compared to conventional cultivation of soya (Leger et al. 2021). New technologies such as CRISPR have greatly increased the potential for optimising SCP production to support a rising demand for protein associated with high land use in agriculture and low conversion efficiency of meat production.
Aquaculture is the fastest-growing food industry in the world and already produces more biomass than either wild seafood or beef (Froehlich et al., 2018). It addresses some key issues of sustainability, but also dilemmas. Fish farming entails significantly lower emissions than ruminant production. Also, aquaculture takes pressure off wild fisheries, vital for the future as over one-third of global wild fisheries have been exploited beyond sustainable limits (FAO, 2020). While the potential for further growth of aquaculture is way above wild fisheries, such expansion will need new sources of quality protein feed with lower environmental impacts than soybean protein (da Silva et al., 2021). Internationally, there are several companies actively pursuing gas fermentation for the production of feed ingredients.
Technologies for capturing and use of atmospheric CO2 (DAC)
In photosynthesis, plants extract and fix CO2 from the air by converting it to biomass with the help of sun energy. In so-called industrial direct air capture (DAC), CO2 is captured and concentrated by industrial processes, either to be utilised (as plants do) or removed from the atmosphere by sequestration (CDR).
Currently, there are two categories of DAC furthest along in development, solid sorbents and liquid solvents (Figure 4.7), while there are other pathways less well developed.
Liquid solvent-based DAC
In the solvent-based approach, gaseous CO2 in air is absorbed into a liquid solvent, resulting in a CO2-depleted gaseous exhaust stream and a CO2-rich liquid exhaust stream. The typical solvent-based approach requires a strong alkaline hydroxide solution to achieve adequate separation of the very dilute CO2 in air. Subsequent anionic exchange results in precipitated calcium carbonate pellets. To complete the cycle, high temperature is used to recover CO2 from the precipitated calcium carbonate and recycling the alkaline reagents. The need to funnel large volumes of air past the absorbing solvent, as well as the regeneration process, drives high energy requirement.
In 2015, Carbon Engineering31, a Canadian company, started operating a pilot plant in Squamish, British Columbia. The pilot captures roughly 1 tonne of atmospheric CO2 per day. Recently, the investment decision was made to construct the world’s first megatonnes DAC facility in Texas in partnership with Occidental 1PointFive and a second plant is undergoing front-end planning and engineering32. This progress is stimulated by the extensive support programme of the Federal US Inflation Reduction Act33. In both Norway and the United Kingdom, a similar DAC facility is being considered, where the CO2 is intended partly for sequestration and partly for use in the production of aviation fuel.
Solid sorbent-based DAC
Solid sorbents have the benefit of the CO2-reactive compound (typically amines) being chemically bound to a solid framework of the air contactor. The desorption and regeneration of the sorbent takes place at a much lower temperature compared to the carbonate-based method described for Carbon Engineering. Moreover, with an operating temperature of 100-120°C, this process may benefit from co-locating with industry offering surplus of heat or the use of geothermal energy exemplified by the Climeworks34 demonstration plant in Iceland (4 kilotonnes per year). This potential energy and cost benefit is possibly countered by a presumed higher CAPEX upon scaling. Interestingly, also Climeworks is engaged in a project in Norway aiming to use DAC for production of aviation fuel.
Figure 4.7. DAC processes combined with CCS and/or CCU

Note: Both Carbon Engineering and Climeworks have CCU projects to produce synthetic fuels. For example, Norsk e-fuel is developing a hybrid technology with Climeworks to take captured CO2 and make a drop-in aviation fuel.
Source: Diagramme from Ozkan et al. (2022).
Carbon sequestration for compensation and removal
Sequestration in building materials
Solid wood construction
The new European Forest Strategy35 of 2021 foresee that forestry could “…contribute to achieving the EU’s biodiversity objectives as well as greenhouse gas emission reduction target of at least 55% by 2030 and climate neutrality by 2050.” There are several ways for forestry to contribute, and one is through increased use of wood and timber in construction to reduce the reliance on concrete. Carbon is sequestered in buildings in this way for many decades or even centuries, and when designed for disassembly and reuse, can be an extremely low-emissions and resilient building material. Skullestad et al. (2016) used LCA to compare timber with concrete and steel as high-rise construction materials with regard to climate change mitigation potential. Timber materials had a significantly lower primary CO2 footprint, and if 90% of timber production residues and timber material waste were incinerated with heat recovery to replace natural gas, a timber structural system can be emissions negative.
Mass timber is a transformative technology made by affixing or gluing together many pieces of wood veneers, flakes or dimension lumber to form larger, stronger pieces of building materials such as solid wood panels, columns or beams that are often used for load-bearing walls, floors and roof construction. There are several types of engineered mass timber products, which are typically categorised by the types of wood products involved and the way they are bound together. The most commonly used types of engineered mass timber products include cross laminated timber (CLT), i.e., large panels of lumber laid flat and combined with alternating direction of the wood fibres. CTL can then be subdivided into nail laminated timber (NLT) and glue laminated timber (GLT) (Figure 4.8).
Figure 4.8. Brock Commons timber-framed high-rise building, Canada

Note: See Canada 1: Embedding carbon in the build environment.
Source: Courtesy of Natural Resources Canada https://natural-resources.canada.ca/home
Engineered mass timber products are engineered for high strength ratings like concrete and steel but are significantly lighter in weight. Prefabrication means that on-site building construction is significantly faster. Moreover, they can be designed specifically for fire resistance, are energy efficient as the low thermal conductivity of wood provides natural insulation that reduces heat loss, and they are well suited to earthquake prone regions due to their durability (Zanuttini and Negro, 2021). Given these functional advantages, combined with the climate benefits, mass timber buildings are slowly being accepted as a sustainable alternative to concrete and steel construction materials (Cabral and Blanchet, 2021).
Injecting CO2 in cement
Portland cement is the most used human-made material (Monteiro et al., 2017). The cement industry has the highest carbon intensity of any industry per unit of revenue and is responsible for approximately 7% of anthropogenic emissions. Unfortunately, concrete is essential also for future construction, and as already discussed, emissions are unavoidable, calling for CCS or other carbon management strategies.
Interestingly, Miller et al (2021) pointed out that cementitious products can absorb and incorporate large amounts of CO2 from many possible sources, thus combining CCU and CCS. Captured CO2 can be reacted with activated minerals or industrial wastes to form stable carbonate minerals. Recent evidence suggests that these minerals create valorisation in their own right, but there is also large potential for their use as supplementary cementitious materials (SCM). Cement replacement such as SCM is the most credible application as others do not match the scale of emissions from cement production and offers several advantages over simple CCS (Skocek et al., 2020). When CO2 is injected into wet concrete, nano-scale calcium carbonate is formed in the concrete as it dries. As well as lowering the carbon footprint, this approach also results in stronger concrete material, which reduces consumption of cement in concrete making. Therefore, the cost of the CO2-containing concrete is cheaper than conventional concrete, which lowers the need for public market incentives.
Geological storage
Sequestering CO2 in deep geological reservoirs is seen as a potential mitigation strategy to reduce anthropogenic CO2 emissions to the atmosphere, and among the most promising of these reservoirs are depleted offshore oil and gas reservoirs (IPCC, 2005). A geological CCS map of the Norwegian part of the North Sea suggests a total storage potential of 70 gigatonnes in this area alone36. As of September 2022, the total global capacity of CCS projects in development was 244 million tonnes per annum of CO2, an increase of 44% over the previous 12 months37.
The IEA has argued consistently that there is no alternative to CCS as the solutions for tackling emissions from heavy industry sectors are often extremely difficult or expensive, hence CCS is an important part of carbon management. However, the Institute for Energy Economics and Financial Analysis (IEEFA) (Robertson and Mousavian, 2022) argue that CCS is a 50 years-old technology and even today the number of success stories is outweighed by failures or underperformance. Clearly there is still work to be done.
While CCS certainly still face challenges, the combination of strengthened climate goals and public programmes, an improved investment environment and new business models have set the stage for greater success in coming years. For more than a decade the number of new projects was low and not encouraging, but 2021 saw a revival (Figure 4.9).
Figure 4.9. Global pipeline of commercial CCUS facilities operating and in development, 2010-2021

Source: IEA, Global pipeline of commercial CCUS facilities operating and in development, 2010-2021, IEA, Paris www.iea.org/data-and-statistics/charts/global-pipeline-of-commercial-ccus-facilities-operating-and-in-development-2010-2021
One of the reasons for this improvement has been policy measures and government initiatives. In the United States the expansion of the 45Q tax credit in 2022, providing a credit of USD 85 per tonne of CO2 from industrial and power facilities that is permanently stored, was a major catalyst for new investment plans. Supported by NOK 18 billion from the government, the Norwegian “Long Ship Project”38 aims to establish the first complete value chain for carbon capture from industry point sources, transporting the CO2 to a centralised hub and storing it permanent below the North Sea. In Australia, AUD 250 million in funding has been announced for CCUS hubs alongside the inclusion of CCUS under the Emissions Reduction Fund39 (with associated credits valued at around AUD 20 per tonne of CO2). The US Inflation Reduction Act of 2022 increased the range of measures available for CCS through 45Q (Box 4.1).
Box 4.1. The US Inflation Reduction Act and DAC
The Inflation Reduction Act of 2022 (IRA) included an historic investment of USD 369 billion in climate and energy funding, as well as important enhancements to the Internal Revenue Service section 45Q on carbon capture and storage. The basis was that global CCS capacity needs to scale from today’s 40 million tonnes per year to multiple gigatonnes per year by 2050, and this law includes provisions that enhance the financial viability of CCS projects in the United States.
The specific 45Q enhancements contained in the IRA include:
Extending the start of construction date from January 2026 to January 2033
Lowering the threshold for captured qualified CO2
Direct Air Capture – 1 000 tonnes
Electricity generating facility – 18 750 tonnes (based on certain design criteria)
Any other industrial facility – 12 500 metric tonnes
Increasing the credit amounts
Point source capture and storage from industrial and power facilities - USD 85 per tonne
Carbon capture and utilisation including enhanced oil recovery - USD 60 per tonne
Direct Air Capture
Capture and storage of carbon – USD 180 per tonne
Capture and utilisation of carbon – USD 130 per tonne
Capture in sediments (between strata)
One of the anxieties around CCS in engineered underground repositories is the potential for escape if the reservoir is not properly sealed. For offshore sites, the impact of any leakage of stored CO2 into the marine environment is not well known or understood. Leakage of CO2 from CCS reservoirs in shallow waters might reduce biodiversity and might also disrupt ecological functions (Lichtschlag et al., 2021).
Injecting CO2 into deep-sea sediments, however, may provide permanent geological storage with fewer worries (Eccles and Pratson, 2012). At the high pressures and low temperatures common in deep-sea sediments, CO2 resides in its liquid phase and can be denser than the overlying pore fluid, making the injected CO2 gravitationally stable (House et al., 2006). This has been carried out safely in Norway for at least 20 years40.
Mineralisation
The earlier mentioned anxiety around CO2 leakage from underground and sub-sea reservoirs is negated in the case of mineralisation41. This is a natural process that can be reproduced artificially. It results in CO2 being converted to solid carbonates, which then cannot re-enter the atmosphere. Mineralisation can be carried out at the surface using already mined rocks, or in deep underground formations, both with different costs. In Iceland the Swiss company Climeworks is storing DAC-derived CO2 as calcite or other carbonate minerals in relatively freshly formed basaltic rocks (Snæbjörnsdóttir and Gislason, 2016).
BECCS and DACCS
Biomass fixes CO2 from the atmosphere during growth and when this biomass is used for energy purposes, as in combustion, it is released again as concentrated CO2. If such biogenic CO2 is captured and stored by geological sequestration or, in the case of biochar formation, applied to land to condition soil, CO2 is removed from the atmospheric cycle. This process is generally referred to as bioenergy with carbon capture and storage (BECCS) or more generally carbon dioxide removal (CDR), involving negative emissions technologies (National Academies of Sciences, Engineering, and Medicine, 2019). Policy would have to drive BECCS with afforestation, in order to make the case for BECCS being truly carbon-negative stronger.
Presumably, due to the need for sustainable procurement of biomass, BECCS deployment is not always treated and/or rewarded in policy and regulations in the same way as emissions sequestered from fossil fuels. For example, at the time of writing, biogenic emissions under Canada’s Output-Based Pricing System (OPBS)42 are exempted from the price on carbon given their carbon neutrality. However, sequestration of these emissions do not generate credits under Canada’s OBPS like sequestration of emissions from fossil fuels. While it does not prohibit BECCS deployment, it does not incentivise the deployment of net-negative technology that could be used to rapidly reduce emissions in heavy emitting sectors. Therefore, treatment of biogenic emissions and net-negative technology will be an important policy and regulatory conversation for carbon management.
Another route to carbon dioxide removal is industrial capturing and concentrating CO2 from ambient air (DAC), as described in Technologies for capturing and use of atmospheric CO2 (DAC). From the scenarios in the fifth assessment report of the IPCC (IPCC, 2014) with a chance of more than 66% to reach the 2°C target, the majority considers directly removing CO2 from ambient air. However, to have any impact, DAC technologies have to be scaled up rapidly. At the time of writing there are 19 plants in operation worldwide, but capturing only about 10 000 tonnes per year, a minute fraction of what is required on the trajectory to net-zero carbon by 2050.
Further technological enablers in carbon-based value chains
Nanotechnology: potential across many CCU areas
Nanotechnology is expected to have a significant impact in all existing industrial sectors, and to harbour the ability to enable the creation of entirely new sectors (OECD, 2017). The main hurdle to achieving commercial-scale production has been insufficient understanding of physical and chemical processes at the nanometre scale, and the inability to control production parameters at that scale, although progress is being made. In this regard, publicly funded cleanroom and nanofabrication infrastructure allows public sector scientists and companies to work together (Figure 4.10).
Figure 4.10. The cleanroom at the LIT Open Innovation Centre (OIC), Austria

Note: The LIT OIC is a collaborative platform where cross-disciplinary academic/scientists and faculty members work alongside company representatives to not only share their expertise and resources, but also create successful synergies. The centre follows a visionary and holistic technological approach with a focus on Responsible Technology. It offers a cleanroom43 and nanofabrication infrastructure which is open for CCUS related projects and activities. The Johannes Keppler University is collaborating in two CCU projects:
1CO2EXIDE (H2020): CO2-based electrosynthesis of ethylene oxide. Objective: The establishment of an electrochemical process for the production of ethylene from CO2, water and renewable energy44.
2ENZYMBIOKAT – Enzymatic bioelectrocatalysis for CO2 reduction. Objective: Conversion of renewable energy (solar and wind) into chemical fuels45.
Source: Photograph courtesy LIT Open Innovation Centre, Austria.
Nanomaterials can be designed to improve the energy efficiency and selectivity of CO2 conversion to fuels and other products, thereby having potential roles in CCU46. One reason is that, as catalysis is very common in industries, nanocatalysis may be used to improve production and energy efficiencies within existing industrial infrastructure. Another is that new nanocatalysts may be deployed that directly convert emissions into useful products and fuels (Mishra et al., 2020).
Dry reforming of methane with CO2 creates a mixture of hydrogen and carbon monoxide (CO) - synthesis gas, or syngas - which can be converted into liquid fuels. It has been a problematic reaction, but a process has been developed using highly stable nanoparticles to overcome operational instabilities (Song et al., 2020). The nanocatalyst is made from inexpensive and abundant materials. Its novelty is that it initiates and speeds up the rate of reaction that converts CO2 and methane into hydrogen gas.
In another example, In2O3 nanoparticles have been demonstrated to selectively catalyse CO2 hydrogenation to green methanol with high stability (Frei et al., 2019). For the reasons detailed in previous sections, methanol is a versatile target for CCU for its roles in chemistry and also as a fuel. Although this and other metal nanocatalysts show promise in the hydrogenation of CO2 to methanol, many barriers still need to be overcome to reach commercialisation (Zheng et al, 2020).
Nanotechnologies have other applications in climate mitigation more generally. Lightweight nanocomposites for materials in transportation save on fuel consumption or improve other properties that lead to higher process efficiency (Graziano et al., 2020). Nano-based lubricants in engines can significantly decrease fuel consumption by reducing friction (Mousavi et al., 2020). Nanocoatings on, say, the surface of aircraft reduce drag and thus reduce fuel consumption47. Cerium oxide nanoparticles as fuel additives aid more complete combustion in engines (Mei et al., 2016). Nanomaterials can help make lighter, stiffer wind turbines (Mishnaevsky Jr et al., 2017). Other applications include photovoltaic technology for solar cells (El Chaar et al., 2011); hydrogen and fuel cells and the hydrogen economy (Sahaym and Norton, 2008); batteries and super-capacitors for energy storage (Gogotsi and Penner, 2018); improved insulation for houses and offices (de Guinoa et al., 2017).
At the cutting edge of nanotechnology/hybrid technologies are nanocell hybrids, which are formed by hybridising abiotic materials with living cells to perform a range of sustainability-driven functions: clean energy, green chemical catalysis, environmental remediation. Nanocell hybrids is a new field that is truly cross-cutting and convergent, encompassing as it does nanotechnology, physics, chemistry, biology, materials science and engineering (Geng et al., 2022).
Nanotechnology in plant agriculture – opportunities and challenges
As an illustration of the general-purpose nature of nanotechnology, Figure 4.11 shows some of the potential applications of nanotechnology in plant agriculture. The authors (Hofmann et al., 2020) assessed that smart delivery of pesticides or nutrients (fertilizers) packaged into nano-carriers are the most mature applications and with the largest potential impact. There are data gaps to be filled, however, to accurately weigh up their risks and benefits. Critical assessment of the market potential and scalability is needed for successful deployment.
Regulation and safety remain critical policy issues and barriers. The engagement of international organisations is required to encourage standardised approaches to nanomaterial regulation. Another barrier is that, unlike other industries for instance pharmaceuticals and automotive, the agricultural industry lacks a unified voice and the resources to develop academic–industry collaborations. And, of course, public acceptance will require engagement of all stakeholders - government regulators, researchers, manufacturers, farmers, consumers and retailers.
Figure 4.11. Nanotechnologies and nanomaterials have applications at many stages in agriculture

Notes: TRL was determined based on the data available on the maturity of the technology, including the scale at which the materials or approach have been applied, the number of studies that provide evidence of efficacy, and the number of commercially available products. TPL was determined based on expert judgment of the potential magnitude of the impacts that each technology may provide to improve agricultural sustainability. Colours indicate the level of opportunity as high (green), medium (yellow) or low (blue). Nc = nano-carrier.
Source: Hofmann et al. (2020)
Automated synthetic chemistry
Compared to the other general-purpose technologies described (synthetic biology/engineering biology, nanotechnology), this is perhaps the least developed. But when perfected, it could herald a “new wave of innovation in biology and materials sciences by greatly facilitating access to known and novel molecules” (Collins et al., 2020). Automated chemical synthesis gets likened to the revolution in genome sequencing, but the main reason that it lags behind biology is that the number of combinations of atoms, particularly in organic molecules, is astronomical, and the technology to build any arbitrary chemical molecule does not yet exist (Sanderson, 2019). While the achievements of modern chemistry are most impressive, current approaches to design and synthesis are still slow and inefficient, with poor reproducibility and scalability, and they make limited use of prior knowledge.
A highly relevant example is a project in automated synthesis to find catalysts that can help to extract hydrogen from water more efficiently. It has taken 20 years of photocatalysis to reach a 1% efficiency in using light to produce hydrogen from water. The goal of the automated synthesis project is to find a material with 5% efficiency, which may mean screening several hundreds of thousands of different molecules. A robotic platform has been built at the University of Liverpool, UK to tackle the task. The ideal automated synthesis platform would be able to plan its own synthetic routes and to execute them, incorporating scale-up to production goals (Coley et al., 2019).
Hydrogen as enabler in carbon-based value chains
In biology, the carbon cycle is the redox process in which carbon oscillates between being bound primarily to oxygen and primarily to hydrogen. Generally, this cycle is driven by sun energy through photosynthesis, whereby atmospheric CO2 and hydrogen, typically from water, is converted into hydrocarbons. Hence, to reduce the pressure on nature by utilising CO2 from industry emissions (CCU) or industrially captured atmospheric CO2 (DAC), hydrogen is a necessary component.
The energy needed to make hydrocarbons is reflected in the fact that the chemical industry is very energy demanding. In a fossil economy, the hydrogen is provided by already existing hydrocarbons such as natural gas or crude oil. To make green alternatives, hydrogen is usually provided as H2 gas, which is a sufficiently energy rich form of hydrogen to react with CO2. However, consistent with common practice, the following discussion refers to H2 simply as hydrogen.
Hence, hydrogen is an essential resource in many carbon-based value chains, not only in CCU and DAC alluded to above, but in a wide spectrum of processes for recycling, upgrading, or converting organic (carbon-based) compounds and materials. This section describes technologies and policies related to hydrogen primarily with reference to manufacturing of carbon products, without downplaying the importance of hydrogen itself as an energy carrier in the energy sector. Hydrogen will also have indirect effects on other value chains, for instance replacing coal as reducing agent in the steel industry or replacing natural gas in the manufacturing of fertilizers.
Production of hydrogen
Figure 4.12 provides an overview of the various production routes to hydrogen, which in industry jargon have been denoted by colours depending on the energy source used. The two most discussed low-emission alternatives are green hydrogen produced from renewable power and blue hydrogen made from natural gas with sequestration of the fossil carbon (CCS). Turquoise hydrogen is a third alternative based on natural gas, where the fossil carbon content is captured on the form of solid carbon (carbon black).
Figure 4.12. The hydrogen economy ‘colours’

Note: United States is moving toward clean hydrogen criteria with well-defined LCA targets versus hydrogen defined by colour.
Source: Adapted from World Energy Council (2021).
Green hydrogen
The generation of green hydrogen is achieved by electrolysis of water powered by renewable energy rather than production from natural gas. Electrolysers consist of an anode and a cathode separated by an electrolyte, much like a fuel cell. Different electrolysers function in different ways, depending on the type of electrolyte material involved and the ionic species it conducts:
Polymer electrolyte membrane (PEM). In this case the electrolyte is a solid speciality plastic material. The special property of this membrane is that it allows passage of protons but not gases such as hydrogen or oxygen, preventing the product gases from mixing.
Alkaline electrolysers. These have been available for many years. Hydroxyl ions (OH-) pass through the electrolyte from the cathode to the anode with hydrogen being generated at the cathode. Research is investigating the use of solid alkaline exchange membranes (AEM).
Solid oxide electrolysers. These use a solid ceramic material as the electrolyte that selectively conducts negatively charged oxygen ions (O2-) from the cathode to the anode, leaving the produced H2 at the cathode.
Hydrogen production via electrolysis using renewable (biomass, wind, solar, hydro, geothermal) or nuclear energy options result in virtually zero GHG emissions but the production cost needs to be decreased significantly to be competitive with more mature pathways based on fossil hydrocarbons48 (grey hydrogen).
Sustainable production of hydrocarbons and derived materials would require emission-free hydrogen. Hence a fundamental consideration for CCU is the hydrogen source and its carbon footprint. If water electrolysis is fuelled only by fossil energy (yellow hydrogen), then it is in fact likely to have an overall carbon footprint larger than conventional grey hydrogen. As most countries still use some fossil resources in their electricity production, the grid power could not be considered fully emission free. Green hydrogen would in such cases either need direct connection to, for instance, a wind park or certification of the sustainability benefits by a third-party LCA.
Blue hydrogen
In principle, the production process for blue hydrogen can be identical to hydrogen produced from natural gas by dry or steam reforming (grey hydrogen), but with the additional step that virtually all the fossil carbon is captured and permanently sequestered. There are also processes in development where the reforming step and the CO2 capture is fully integrated (Andresen et al., 2014). In practice, however, the complete CCS process is not 100%. Although the carbon footprint may potentially be lower than 1 kg CO2e per kg hydrogen produced (DNV, 2021), blue hydrogen is classified as “low-emission hydrogen” in the EU nomenclature, in contrast to green hydrogen which can be “renewable” provided that the electrical power is fully renewable.
Blue hydrogen depends on access to natural gas, as well as opportunities for CO2 sequestering. The US Inflation Reduction Act offers public support for building CCS sites linked to natural gas wells and tax incentives for use of blue hydrogen. In Norway there are plans for blue hydrogen production at the current oil and gas refinery at Mongstad, which is only 40 kilometres from the Norther Lights CCS hub. However, hydrogen is difficult to transport over long distances, hence for central European markets it might be better to transport natural gas from the North Sea in existing pipelines, extract the hydrogen close to its use, and then transport the CO2 back for storage, rather than transporting the final hydrogen product to the market.
National hydrogen strategies
Given the general utility and importance of hydrogen in a net-zero scenario, many countries have developed a dedicated hydrogen strategy. Table 4.2 provides an overview of several OECD member states that have already a national strategy in place, while others are in the process of doing so.
Table 4.2. National hydrogen strategies of selected OECD countries
Country and year |
Source |
Policy implications |
---|---|---|
Australia, 2019 |
www.industry.gov.au/data-and-publications/australias-national-hydrogen-strategy |
Rapid scale-up strategy; Identifies 57 joint actions, Capacity building; ‘Responsive’ regulation; Industrial feedstock; Heating; Heavy transport; Lon-term governance structures; Safety |
Canada, 2020 |
www.nrcan.gc.ca/climate-change/canadas-green-future/the-hydrogen-strategy/23080 |
New H2 supply, distribution infrastructure; Drive investment through regulation; Key enabler for net-zero emissions, 2050; Engage indigenous communities; Create 250 000 jobs; Energy resilience |
Chile, 2020 |
https://energia.gob.cl/sites/default/files/national_green_hydrogen_strategy_-_chile.pdf |
Emissions reduction; Greening industry; Local jobs; Public-private partnerships; Create electrolysis sector; Energy exports; Competitive production; 6 priority applications; Standards, safety; Governance |
France, 2020 |
www.economie.gouv.fr/presentation-strategie-nationale-developpement-hydrogene-decarbone-france |
R&D&I; Scale-up barriers; Electrolyser sector; Heavy transport; Skills; Energy security; Local; Regional; 11 key markets; Clean mobility; Jobs; Deployment; Decarbonise; Defossilise; Europe; Infrastructure |
Germany, 2020 |
www.bmwi.de/Redaktion/EN/Publikationen/Energie/the-national-hydrogen-strategy.html |
Market creation; Technology rollout; Offshore and onshore; Security; Alternative energy; Chemicals, steel sectors; transport infrastructure; Skilled labour; International markets; Create National Hydrogen Council |
Hungary, 2021 |
https://cdn.kormany.hu/uploads/document/a/a2/a2b/a2b2b7ed5179b17694659b8f050ba9648e75a0bf.pdf |
Action plan; Target system; Decentralised; Industry decarbonisation; Electrolyser capacity building; Green transport; Electricity infrastructure; Regulation, International cooperation, R&D&I, Skills, education; |
Italy, 2021 |
Electrolyser capacity; Green H2; 10 000 jobs; Transport; Chemicals; Market costs; Emissions; Regulation; Standards; Investments; Refineries, Ammonia, Iron/steel, H2 corridors; Infrastructure; R&I |
|
Japan, 2017 |
Cost-competitive with fossil; Energy security; Emissions reduction; Heat; Power; Industry processes; Transport; Safety, Supply chains; Pipelines technology; Standards; International cooperation; Public support |
|
Korea, 2019 |
www.korea.kr/special/policyCurationView.do?newsId=148857966#L4 |
Scale-up; Vehicles; Power; Heating; Imports; Economic growth; Safety; Regulation; R&D; Standards; Market; Financing and investments; Competitiveness; Energy security; Roadmap; Infrastructure |
Netherlands, 2020 |
www.government.nl/documents/publications/2020/04/06/government-strategy-on-hydrogen |
National Climate Agreement; Clean H2; Job creation; Scale-up; Cost reduction; PPP; Global market; Air quality; Supply chain; Clusters; Regulation; Safety; R&D&I; Regional; International strategy |
Norway, 2020 |
www.regjeringen.no/contentassets/8ffd54808d7e42e8bce81340b13b6b7d/hydrogenstrategien-engelsk.pdf |
Value creation; CO2 tax; Demonstration; Piloting; Value chain; CCS; Electrolysis; Competitive; Safety; Standards; Zero Emissions Fund; Maritime; Public procurement; International collaboration; ETS |
United States |
www.hydrogen.energy.gov/clean-hydrogen-strategy-roadmap.html |
Scale-up; Safe storage; Levelised cost; Permitting; Decarbonisation; Supply chain resilience; Codes; Standards; Jobs; Training; Private investment; Chemicals; Steelmaking; Transport; Electricity; RDD&D; Holistic; Justice; Regional hubs; Electolysers, Regulation |
European Union, 2020 |
https://ec.europa.eu/energy/sites/ener/files/hydrogen_strategy.pdf |
Emissions reduction; Air quality; Achieve Green Deal; Decarbonise; Deployment, Investment; Electrolysers; Lead markets; PPPs; Market; Carbon capture; Investment; Clean Hydrogen Alliance; Roadmap 2050; |
Note: The selected policy implications for each country are not exhaustive. They demonstrate the range of policy issues at stake and highlight commonalities and differences.
One of the general observations from the national strategies is that many envisage multiple roles for hydrogen, although the timescales for deployment vary (Table 4.3). A quite common objective is to provide the means to reach national climate obligations, for instance low-emission solutions in the transport sector. This may include using hydrogen directly in a fuel cell or indirectly by CCU-based production of so-called synthetic fuels for heavy vehicles, aviation or the maritime sector. Other aspects may be related to sustainability goals in specific domestic industry sectors, such as steel, cement, ammonia, or organic chemicals.
Table 4.3. Sectors prioritised in national hydrogen strategies in selected OECD member states
Sector |
Priority in National Hydrogen Strategy |
||||||||||
---|---|---|---|---|---|---|---|---|---|---|---|
Australia |
Canada |
Chile |
France |
Germany |
Hungary |
Japan |
Korea |
Netherlands |
Norway |
European Union |
|
Heating |
Immediate |
Immediate |
Immediate |
Lower |
Lower |
Immediate |
Immediate |
Lower |
Immediate |
Lower |
Lower |
Industry: |
|||||||||||
Iron/steel |
Long term |
Immediate |
N/A |
Immediate |
Immediate |
Long term |
Lower |
Lower |
Immediate |
Lower |
Long term |
Chemical feedstock |
Immediate |
Immediate |
Immediate |
Immediate |
Immediate |
Immediate |
Lower |
N/A |
Immediate |
Immediate |
Immediate |
Refining |
N/A |
Immediate |
Immediate |
Immediate |
Immediate |
Immediate |
Lower |
N/A |
Immediate |
Lower |
Immediate |
Other (e.g. cement) |
N/A |
Immediate |
N/A |
Immediate |
Lower |
Long term |
N/A |
N/A |
Lower |
N/A |
N/A |
Power generation |
Lower |
Lower |
N/A |
N/A |
N/A |
Lower |
Immediate |
Immediate |
Lower |
N/A |
Lower |
Transport: |
|||||||||||
Light vehicles |
Lower |
Immediate |
Long term |
Lower |
Lower |
Long term |
Immediate |
Immediate |
Immediate |
Lower |
Lower |
Medium, heavy |
Immediate |
Immediate |
Immediate |
Immediate |
Immediate |
Immediate |
Long term |
Immediate |
Immediate |
Lower |
Immediate |
Buses |
Immediate |
Immediate |
Immediate |
Immediate |
Immediate |
Immediate |
Long term |
Immediate |
Immediate |
Lower |
Immediate |
Rail |
Lower |
Long term |
N/A |
Immediate |
Immediate |
Lower |
Lower |
Lower |
Immediate |
N/A |
Immediate |
Marine |
Long term |
Long term |
Long term |
Lower |
Long term |
Lower |
Lower |
Lower |
Lower |
Immediate |
Long term |
Aviation |
Lower |
Long term |
Long term |
Immediate |
Long term |
N/A |
Lower |
N/A |
Lower |
Lower |
Long term |
Note: N/A = not applicable
Source: Adapted from World Energy Council (2021)
Hydrogen policies for chemicals, materials, and fuels
The policy analysis provided in Table 4.3 shows the importance of hydrogen in manufacturing of chemicals. Saygin and Gielen (2021) argued that green hydrogen is essential for the chemicals industry to reach 2050 targets. On the basis of this analysis, chemical feedstock is jointly the top sector where ‘immediate’ action is needed.
While the technology for both green and blue hydrogen already exist, the current global production is still low, about 3 megatonnes of blue and only 260 kilotonnes of green hydrogen49 of a total demand of 15 megatonnes. As alluded to, the current costs of both green and blue hydrogen are considerably higher than for traditional ‘grey’ hydrogen. Cost-competitiveness is a major issue today and for some time into the future. Driving down costs will require a range of supply- and demand-side instruments. On the demand-side, markets need to be incentivised and developed. That will require standards and robust regulation and governance. Green procurement is another market-based mechanism of interest.
Electrolysis makes it feasible for multiple countries to produce their own hydrogen. It is inevitable, however, that some countries will have national advantages, although the success formula can be complex. Countries in the Middle East may for instance benefit from an ample supply of relatively cheap sun energy but will also need stoichiometric amounts of pure freshwater not so easily available.
Apart from the cost, the availability of electricity for green hydrogen production will have to compete with alternative use of the renewable power. If a country has a plan to build economic growth around CCU and does not have the renewable energy capacity, the cost of infrastructure for hydrogen import must be included, which can be considerable. Gas-for-Climate (2021) is an informative document describing hydrogen infrastructure and financing plans in various European countries.
As synthetic chemicals and fuels require large investments, a clear regulatory framework is essential, particularly with respect to demand-side measures. This is illustrated by the efforts to stimulate sustainable aviation fuel (SAF) in Europe. As part of their “Fit For 55” programme, the European Commission has announced ambitious blending mandates, rising from 2% SAF in 2025 to 20% in 2035 and 70% in 205050 (Refuel EU). Of this there is a proposed sub-mandate of synthetic SAF (35% in 2050), which would depend on hydrogen. However, to qualify as synthetic SAF, only green hydrogen made from fully renewable energy would be allowed51.
Korea acts as an exemplar of policy to develop a hydrogen ecosystem. For Korea, the ambitions are large and a little different from other countries in the emphasis on transportation and power generation (Box 4.2). It also illustrates the need to align demand side ambitions with realistic supply side opportunities.
Box 4.2. Korea’s hydrogen economy policy
For much of this century so far Korea has been investing in hydrogen through both the public and private sectors, resulting in a maturing industry targeting mobility and power generation in near term. This has been achieved by a policy mix designed to attract many companies that have formed the Korean hydrogen ecosystem. It is estimated that there are almost 400 companies in the Korean hydrogen industry.
Korea’s hydrogen policy portfolio
Master Plan for an Environmentally Friendly Hydrogen Economy—Visions (2005)
This set targets of 3% for the share of hydrogen in final energy, supply of two million hydrogen vehicles (8% of all vehicles), and 6.8% for the share of fuel cells for power generation in total power demand. Korea proved ahead of its time, and the plan never met its targets, one reason being a lack of international interest.
Strategic Investment Direction for Innovative Growth (2018)
The Korean government selected the hydrogen economy as one of three major strategic investment areas.
Hydrogen Economy Activation Roadmap (2019)
During 2018 a national committee prepared a roadmap, which was launched in 2019, establishing three main policy directions to realise the hydrogen economy:
1. Create an industrial ecosystem around hydrogen vehicles and fuel cells.
2. Build a production capacity of 5 million tonnes hydrogen by 2040.
3. To prepare and implement safety management standards for hydrogen.
Act on Hydrogen Economy Development and Hydrogen Safety Management
The Hydrogen Act makes provisions for hydrogen equipment safety requirements, certification processes and the roles and responsibilities of various government agencies. The controlling body is the Hydrogen Economy Committee chaired by the Prime Minister and with ministers from eight ministries.
Green New Deal (2020)
Out of the KRW 74 trillion (USD 56 billion) of total capital investment under the Green New Deal, the largest portion, KRW 20 trillion, is to be used for green mobility, particularly hydrogen projects.
Source: Department of International Trade (UK) (2021); Youngok et al. (2022)
References
Anderson, J.A., P.C. Ellsworth, J.C. Faria, G.P. Head, M.D.K. Owen, C.D. Pilcher, A.M. Shelton and M. Meissle (2019), “Genetically engineered crops: importance of diversified integrated pest management for agricultural sustainability”, Frontiers in Bioengineering and Biotechnology 7, https://doi.org/10.3389/fbioe.2019.00024.
Andersson, K. and C.M. Salazar (2015), “Methanol as a marine fuel; report”, Methanol Institute: Brussels, Belgium.
Andresen, B., A. Norheim, J. Strand, Ø. Ulleberg, A. Vik and I. Wærnhus (2014), “BioZEG – pilot plant demonstration of high efficiency carbon negative energy production”, Energy Procedia 63, 279-285.
Baveye, P.C., J. Baveye and J. Gowdy (2016), “Soil “ecosystem” services and natural capital: critical appraisal of research on uncertain ground”, Frontiers in Environmental Science 4:41.
Beh, B. and M. Dent (2022), “Vertical Farming 2022-2032. Controlled Environment Agriculture including hydroponics, aeroponics, aquaponics, automation, sensors, LEDs, and container farms. Analysis by region and business model. Forecast of produce by region”, ISBN 9781915514028, www.idtechex.com/en/research-report/vertical-farming-2022-2032/871.
Bell, J., J. Philp and R.I. Kitney, R.I. (2021) “Addressing the post-COVID era through engineering biology”, Engineering Biology 5, 21-34.
Brownlie, W.J., M.A. Sutton, K.V. Heal, D.S. Reay and B.M. Spears. (eds.) (2022), “Our Phosphorus Future”, UK Centre for Ecology and Hydrology, Edinburgh. doi: 10.13140/RG.2.2.17834.08645.
Budhlakoti, N., A.K. Kushwaha, A. Rai, K.K. Chaturvedi, A. Kumar, A.K. Pradhan, U. Kumar, R.R. Kumar, P. Juliana, D.C. Mishra and S. Kumar (2022), “Genomic selection: a tool for accelerating the efficiency of molecular breeding for development of climate-resilient crops”, Frontiers in Genetics 13:832153.
Cabral, M.R. and P.A. Blanchet (2021), “State of the art of the overall energy efficiency of wood buildings—an overview and future possibilities”, Materials 14:1848.
Carus, M., L. Dammer, A. Raschka and P. Skoczinski (2020), “Renewable carbon: Key to a sustainable and future-oriented chemical and plastic industry: Definition, strategy, measures and potential”, Greenhouse Gases Science and Technology 10, 488-505.
Coban, O., G.B. De Deyn and M. Van Der Ploeg (2022), “Soil microbiota as game-changers in restoration of degraded lands”, Science 375:eabe0725.
Coley, C.W., D.A. Thomas III, J.A.M. Lummiss, J.N. Jaworski, C.P. Breen, V.T. Hart, J.S. Fishman, L. Rogers, H. Gao, R.W. Hicklin, P.P. Plehiers, J. Byington, J.S. Piotti, W.H. Green, A.J. Hart, T.F. Jamison and K.F. Jensen (2019), “A robotic platform for flow synthesis of organic compounds informed by AI planning”, Science 365:eaax1566.
Collins, N., D. Stout, J.-P. Lim, J.P. Malerich, J.D. White, P.B. Madrid, M. Latendresse, D. Krieger, J. Szeto, V.-A. Vu, K. Rucker, M. Deleo, Y. Gorfu, M. Krummenacker, L.A. Hokama, P. Karp and S. Mallya (2020), “Fully automated chemical synthesis: toward the universal synthesizer” Organic Process Research and Development 24, 2064-2077.
Cook, B.I., T.R. Ault and J.E. Smerdon (2015), “Unprecedented 21st century drought risk in the American Southwest and Central Plains”, Science Advances 1:e1400082.
da Silva, R.F.B., A. Viña, E.F. Moran, Y. Dou, M. Batistella and J. Liu (2021), “Socioeconomic and environmental effects of soybean production in metacoupled systems”, Scientific Reports 11:18662.
de Guinoa, A.S., D. Zambrana-Vasquez, A. Alcalde, M. Corradini and I. Zabalza-Bribián (2017), “Environmental assessment of a nano-technological aerogel-based panel for building insulation”, Journal of Cleaner Production 161, 1404-1415.
DeLisi, C. (2019), “The role of synthetic biology in climate change mitigation”, Biology Direct 14:14, https://doi.org/10.1186/s13062-019-0247-8.
Department of International Trade (UK) (2021), “The Hydrogen Economy South Korea. Market Intelligence Report”, January 2021, Department of International Trade, London.
De Souza, A.P., S.J. Burgess, L. Doran, J. Hansen, L. Manukyan, N. Maryn, D. Gotarkar, L. Leonelli, K.K. Niyogi and S.P. Long (2022), “Soybean photosynthesis and crop yield are improved by accelerating recovery from photoprotection”, Science 377, 851-854.
D’Hondt, K., G. Jiménez-Sánchez, G. and J.C. Philp (2015), “Reconciling agricultural and industrial needs for an Asian bioeconomy”, Asian Biotechnology and Development Review 17, 85-130.
D’Hondt, K., T. Kostic, R. McDowell, F. Eudes, B.K. Singh, S. Sarkar, M. Markakis, B. Schelkle, E. Maguin and A. Sessitsch (2021), “Microbiome innovations for a sustainable future”, Nature Microbiology 6, 138-142.
DNV (2021), “Blue hydrogen in a low-carbon energy future. Exploring what it takes to produce low-carbon blue hydrogen”, DNV AS, Hovik, Norway.
Eccles, J.K. and L. Pratson (2012), “Global CO2 storage potential of self-sealing marine sedimentary strata”, Geophysical Research Letters 39:L19604.
El Chaar, L., L.A. Lamont and N. El Zein (2011), “Review of photovoltaic technologies”, Renewable and Sustainable Energy Reviews 15, 2165-2175.
Erickson, E.D., P.A. Tominac and V.M. Zavala (2023), “Biogas production in United States dairy farms incentivized by electricity policy changes”, Nature Sustainability, https://doi.org/10.1038/s41893-022-01038-9.
Eriksen, M., W. Cowger, L.M. Erdle, S. Coffin, P. Villarrubia-Gómez, C.J. Moore, E.J. Carpenter, R.H. Day, M. Thiel and C. Wilcox (2023), “A growing plastic smog, now estimated to be over 170 trillion plastic particles afloat in the world’s oceans—Urgent solutions required”, PLoS ONE 18: e0281596.
European Commission (2018), “A European strategy for plastics in a circular economy”, COM(2018) 28 final, European Commission, Brussels.
European Commission (2007), “Environment Fact Sheet: Soil protection – A new policy for the EU”. (KH-15-04-014-EN-C).
FAO (2020). The state of world fisheries and aquaculture 2020. Sustainability in action. UN Food and Agriculture Organization, Rome.
Foyer, C.H., A.V. Ruban and P.J. Nixon (2017), “Photosynthesis solutions to enhance productivity”, Philosophical Transactions of the Royal Society B 372:20160374.
Fraser, E.D.G. and M. Campbell (2019), “Agriculture 5.0: reconciling production with planetary health”, One Earth 1, 278-280.
Frei, M.S., C. Mondelli, A. Cesarini, F. Krumeich, R. Hauert, J.A. Stewart, D. Curulla Ferré and J. Pérez-Ramírez (2019), “Role of zirconia in indium oxide-catalyzed CO2 hydrogenation to methanol”, ACS Catalysis 10, 1133–1145.
French, K.E. (2019), “Harnessing synthetic biology for sustainable development”, Nature Sustainability 2, 250-252.
Froehlich, H.E., C.A. Runge, R.R. Gentry, S.D. Gaines and B.S. Halpern (2018), “Comparative terrestrial feed and land use of an aquaculture-dominant world”, Proceedings of the National Academy of Sciences of the United States of America 115, 5295-5300.
Gas-for-Climate (2021), “Priorities for the EU hydrogen legislation”, https://gasforclimate2050.eu/wp-content/uploads/2021/06/Gas-for-Climate-Priorities-for-the-EU-hydrogen-legislation-24-June-2021-2.pdf.
Geng, W., L. Wang, and X.-Y. Yang (2022), “Nanocell hybrids for green chemistry”, Trends in Biotechnology 40, 974-986.
Geyer, R., J.R. Jambeck and K.L. Law (2017), “Production, use, and fate of all plastics ever made”, Science Advances 3:e1700782.
Gogotsi, Y. and R.M. Penner (2018), “Energy storage in nanomaterials – capacitive, pseudocapacitive, or battery-like?”, ACS Nano 12, 2081-2083.
Gore, A. (2013), “The future: Six drivers of global change”, Random House, New York.
Graziano, G., C. Garcia, S. Jaffer, J. Tjong, W. Yang and M. Sain (2020), “Functionally tuned nanolayered graphene as reinforcement of polyethylene nanocomposites for lightweight transportation industry”, Carbon 169, 99-110.
Groenewald, M., T. Boekhout, C. Neuvéglise, C. Gaillardin, P.W.M. van Dijck and M. Wyss (2014), “Yarrowia lipolytica: safety assessment of an oleaginous yeast with a great industrial potential”, Critical Reviews in Microbiology 40, 187–206.
Gullino, M.L, R. Albajes, I. Al-Jboory, F. Angelotti, S. Chakraborty, K,A. Garrett, B.P. Hurley, P. Juroszek, R. Lopian, K. Makkouk, X. Pan, M. Pugliese and T. Stephenson (2022), “Climate change and pathways used by pests as challenges to plant health in agriculture and forestry” Sustainability 14, 12421.
Handelsman, J. (2021), “A world without soil: The past, present, and precarious future of the Earth beneath our feet”, Yale University Press, New Haven, Connecticut.
Handler, R.M., D.R. Shonnard, E.M. Griffing, A. Lai and I. Palou-Rivera (2016), “Life Cycle Assessments of ethanol production via gas fermentation: anticipated greenhouse gas emissions for cellulosic and waste gas feedstocks”, Industrial and Engineering Chemistry Research 55, 3253-3261.
Hefferon, K.L. (2015), “Nutritionally enhanced food crops; progress and perspectives”, International Journal of Molecular Sciences 16, 3895-3914.
Hepburn, C., E. Adlen, J. Beddington, E.A. Carter, S. Fuss, N. MacDowell, J.C. Minx, P. Smith, C.K. Williams (2019), “The technological and economic prospects for CO2 utilization and removal”, Nature 575, 87-97.
Hofmann, T. G.V. Lowry, S. Ghoshal, N. Tufenkji, D. Brambilla, J.R. Dutcher, L.M. Gilbertson, J.P. Giraldo, J.M. Kinsella, M.P. Landry, W. Lovell, R. Naccache, M. Paret, J.A. Pedersen, J.M. Unrine, J.C. White and K.J. Wilkinson (2020), “Technology readiness and overcoming barriers to sustainably implement nanotechnology-enabled plant agriculture”, Nature Food 1, 416-425.
House, K.Z., D.P. Schrag, C.F. Harvey and K.S. Lackner (2006), “Permanent carbon dioxide storage in deep-sea sediments”, Proceedings of the National Academy of Science of the United States of America 103, 12291-12295.
IEA (2021), “Carbon capture in 2021: Off and running or another false start?”, IEA Publishing, Paris.
IEA Bioenergy (2015), “Exploring the viability of small scale anaerobic digesters in livestock farming”, ISBN 978-1-910154-25-0.
IEA, ICCA, DECHEMA. (2013), “Technology roadmap – energy and GHG reductions in the chemical industry via catalytic processes”, https://iea.blob.core.windows.net/assets/d0f7ff3a-0612-422d-ad7d-a682091cb500/TechnologyRoadmapEnergyandGHGReductionsintheChemicalIndustryviaCatalyticProcesses.pdf.
IPCC (2020), “Summary for Policy makers. In: Climate Change and Land: an IPCC special report on climate change, desertification, land degradation, sustainable land management, food security, and greenhouse gas fluxes in terrestrial ecosystems”, P.R. Shukla, J. Skea, E. Calvo Buendia, V. Masson-Delmotte, H.- O. Pörtner, D. C. Roberts, P. Zhai, R. Slade, S. Connors, R. van Diemen, M. Ferrat, E. Haughey, S. Luz, S. Neogi, M. Pathak, J. Petzold, J. Portugal Pereira, P. Vyas, E. Huntley, K. Kissick, M. Belkacemi, and J. Malley, (eds.). Intergovernmental Panel on Climate Change (IPCC), Geneva, Switzerland.
IPCC (2014), “Climate change 2014: synthesis report. Contribution of working groups I, II and III to the fifth assessment report of the intergovernmental panel on climate change”, Intergovernmental Panel on Climate Change (IPCC), Geneva, Switzerland.
IPCC (2005), “Special report on carbon dioxide capture and storage”, Prepared by Working Group III of the Intergovernmental Panel on Climate Change, IPCC. Cambridge University Press, Cambridge, UK.
IUCN (2021), “Issues Brief. Marine plastic pollution”, International Union for Conservation of Nature, Gland, Switzerland. www.iucn.org/resources/issues-brief/marine-plastic-pollution#:~:text=Over%20400%20million%20tons%20of,waters%20to%20deep%2Dsea%20sediments.
Jansson, J.K. and K.S. Hofmockel (2020), “Soil microbiomes and climate change”, Nature Reviews Microbiology 18, 35-46.
Jasi, A. (2022), “Novel method for green ammonia production”, The Chemical Engineer, February 2022.
Jones, S.W., A. Karpol, S. Friedman, B.T. Maru and B.P. Tracy (2020). Recent advances in single cell protein use as a feed ingredient in aquaculture. Current Opinion in Biotechnology 61, 189-197.
Karlen, D.L. and C.W. Rice (2015), “Soil degradation: Will humankind ever learn?”, Sustainability 7/9, 12490-12501.
Kinley, R.D., G. Martinez-Fernandez, M.K. Matthews, R. de Nys, M. Magnusson, N.W. Tomkins (2020), “Mitigating the carbon footprint and improving productivity of ruminant livestock agriculture using a red seaweed”, Journal of Cleaner Production 259, 120836.
Kitney, R., M. Adeogun, Y. Fujishima, Á. Goñi-Moreno, R., Johnson, M. Maxon, S. Steedman, S. Ward, D. Winickoff and J. Philp (2019), “Enabling the advanced bioeconomy with engineering biology”, Trends in Biotechnology 37, 917-920.
Kromdijk J., K. Głowacka, L. Leonelli, S.T. Gabilly, M. Iwai, K.K. Niyogi and S.P. Long (2017), “Improving photosynthesis and crop productivity by accelerating recovery from photoprotection”, Science 354, 857-860.
Lamba P., D.P. Kaur, S. Raj and J. Sorout (2021), “Recycling/reuse of plastic waste as construction material for sustainable development: a review”, Environmental Science and Pollution Research, https://doi.org/10.1007/s11356-021-16980-y.
Lassen, J. and G.F. Difford (2020), “Review: Genetic and genomic selection as a methane mitigation strategy in dairy cattle”, Animal 14:S3, s473–s483.
Leger, D., S. Matassa, E. Noor, A. Shepon, R. Miloc and A. Bar-Even (2021), “Photovoltaic-driven microbial protein production can use land and sunlight more efficiently than conventional crops” Proceedings of the National Academy of Sciences of the United States of America 118, 26:e2015025118.
Liakakou, E.T., A. Infantes, A. Neumann and B.J. Vreugdenhil (2021), “Connecting gasification with syngas fermentation: Comparison of the performance of lignin and beech wood”, Fuel 290: 120054.
Licht, S., B. Cui, B. Wang, F.-F. Li, J. Lau and S. Liu (2014), “Ammonia synthesis by N2 and steam electrolysis in molten hydroxide suspensions of nanoscale Fe2O3”, Science 345, 637-640.
Lichtschlag, A., M. Haeckel, D. Olierook, K. Peel, A. Flohr, C.R. Pearce, C. Marieni, R.H. James and D.P. Connelly (2021), “Impact of CO2 leakage from sub-seabed carbon dioxide storage on sediment and porewater geochemistry”, International Journal of Greenhouse Gas Control 109, 03352.
Liew, F., M.E. Martin, R.C. Tappel, B.D. Heijstra, C. Mihalcea and M. Köpke (2016), “Gas fermentation – A flexible platform for commercial scale production of low-carbon-fuels and chemicals from waste and renewable feedstocks”, Frontiers in Microbiology 7:694, https://doi.org/10.3389/fmicb.2016.00694
Liew, F.E., Nogle, R., Abdalla, T. et al. (2022), Carbon-negative production of acetone and isopropanol by gas fermentation at industrial pilot scale. Nat Biotechnol 40, 335–344. https://doi.org/10.1038/s41587-021-01195-w.
Maleki, F., M. Changizian, S. Zolfaghari, S. Rajaei, K.A. Noghabi and H.S. Zahiri (2021), “Consolidated bioprocessing for bioethanol production by metabolically engineered Bacillus subtilis strains”, Scientific Reports 11:13731.
Massé, D.I., G. Talbot and Y. Gilbert (2011), “On farm biogas production: A method to reduce GHG emissions and develop more sustainable livestock operations”, Animal Feed Science and Technology 166-167, 436-445.
Mei, D., X. Li, Q. Wu and P. Sun (2016), “Role of cerium oxide nanoparticles as diesel additives in combustion efficiency improvements and emission reduction”, Journal of Energy Engineering 142: 04015050.
Mihaila, A.A., C.R.K. Glasson, R. Lawton, S. Muetzel, G. Molano and M. Magnusson (2022), “New temperate seaweed targets for mitigation of ruminant methane emissions: an in vitro assessment”, Applied Phycology 3, 274-284.
Miller, S.A., G. Habert, R.J. Myers, and J.T. Harvey (2021), “Achieving net zero greenhouse gas emissions in the cement industry via value chain mitigation strategies”, One Earth 1398-1411.
Mishnaevsky Jr, L., K. Branner, H.N. Petersen, J. Beauson, M. McGugan and B.F. Sørensen (2017), “Materials for wind turbine blades: An overview”, Materials 10:1285.
Mishra, A.K., R. Belgamwar, R. Jana, A. Datta and V. Polshettiwar (2020), “Defects in nanosilica catalytically convert CO2 to methane without any metal and ligand”, Proceedings of the National Academy of Sciences of the United States of America 117, 6383-6390.
Monteiro, P.J.M., S.A. Miller and A. Horvath (2017), “Towards sustainable concrete”, Nature Materials 16, 698-699.
Mousavi, S.B., S.Z. Heris and P. Estellé (2020), “Experimental comparison between ZnO and MoS2 nanoparticles as additives on performance of diesel oil-based nano lubricant”, Scientific Reports 10: 5813.
Nannipieri, P., C.R. Penton, W. Purahong, M. Schloter and J.D. van Elsas (2019), “Recommendations for soil microbiome analyses”, Biology and Fertility of Soils 55, 765-766.
National Academies of Sciences, Engineering, and Medicine (2019), “Negative emissions technologies and reliable sequestration. A research agenda”, The National Academies Press, Washington DC.
Northrup, D.L., B. Basso, M.Q. Wang, C.L. Morgan and P.N. Benfey (2021), “Novel technologies for emission reduction complement conservation agriculture to achieve negative emissions from row-crop production”, Proceedings of the National Academy of Sciences, 118(28), e2022666118.
Obšteter, J., J. Jenko and G. Gorjanc (2021), “Genomic selection for any dairy breeding program via optimized investment in phenotyping and genotyping”, Frontiers in Genetics 12:637017.
O’Connor, S., E. Ehimen, S.C. Pillai, A. Black, D. Tormey and J. Bartlett (2021), “Biogas production from small-scale anaerobic digestion plants on European farms”, Renewable and Sustainable Energy Reviews 139:110580.
OECD (2022), “Global Plastics Outlook: economic drivers, environmental impacts and policy options”, OECD Publishing, Paris.
OECD (2017), “The next production revolution: implications for governments and business”, OECD Publishing, Paris.
OECD (2009), “The Bioeconomy to 2030: designing a policy agenda”, OECD Publishing, Paris.
Ozkan, M., S.P. Nayak, A.D. Ruiz and W. Jiang (2022), “Current status and pillars of direct air capture technologies”, iScience 25:103990.
Paine, J., C. Shipton, S. Chaggar, S., R.M. Howells, M.J. Kennedy, G. Vernon, S.Y. Wright, E. Hinchliffe, J.L. Adams, A.L. Silverstone and R. Drake (2005), “Improving the nutritional value of Golden Rice through increased pro-vitamin A content”, Nature Biotechnology 23, 482-487.
Pander, B., Z. Mortimer, C. Woods, C. McGregor, A. Dempster, L. Thomas, J. Maliepaard, R. Mansfield, P. Rowe and P. Krabben (2020), “Hydrogen oxidising bacteria for production of single-cell protein and other food and feed ingredients”, Engineering Biology 4, 21-24.
Poore, J. and T. Nemecek (2018), “Reducing food’s environmental impacts through producers and consumers”, Science 360, 987-992.
Robertson, B. and M. Mousavian (2022), “The carbon capture crux: Lessons learned”, Institute for Energy Economics and Financial Analysis (IEEFA), Lakewood, OH, United States. https://ieefa.org/resources/carbon-capture-crux-lessons-learned.
Rosenblueth, M., E. Ormeño-Orrillo, A. López-López, M.A. Rogel, B.J. Reyes-Hernández, J.C. Martínez-Romero, P.M. Reddy, and E. Martínez-Romero (2018), “Nitrogen fixation in cereals”, Frontiers in Microbiology 9:1794.
Royal Society Policy Briefing (2020), “Ammonia: zero-carbon fertilizer, fuel and energy store”, The Royal Society, London, ISBN: 978-1-78252-448-9.
Sahaym, U. and M.G. Norton (2008), “Advances in the application of nanotechnology in enabling a ‘hydrogen economy’”, Journal of Material Science 43, 5395-5429.
Sanderson, K. (2019), “Automation: Chemistry shoots for the moon”, Nature 568, 755-579.
Saygin, D. and D. Gielen (2021), “Zero-emission pathway for the global chemical and petrochemical sector”, Energies 14, 3772, https://doi.org/10.3390/en14133772.
Schmidt, H.-P., A. Anca-Couce, N. Hagemann, C. Werner, D. Gerten, W. Lucht and C. Kammann (2019), “Pyrogenic carbon capture and storage”, GCB Bioenergy 11, 573-591.
Schorn, F., J.L. Breuer, R.C. Samsun, T. Schnorbus, B. Heuser, R. Peters and D. Stolten (2021), “Methanol as a renewable energy carrier: An assessment of production and transportation costs for selected global locations”, Advances in Applied Energy 3:100050.
Schuur, E., A. McGuire, C. Schädel, G. Grosse, J.W. Harden, D.J. Hayes, G. Hugelius, C.D. Koven, P. Kuhry, D.M. Lawrence, S.M. Natali, D. Olefeldt, V.E. Romanovsky, K. Schaefer, M.R. Turetsky, C.C. Treat and J.E. Vonk (2015), “Climate change and the permafrost carbon feedback”, Nature 520, 171-179.
Schwarzenbeck, N., W. Pfeiffer and E. Bomball (2008), “Can a wastewater treatment plant be a powerplant? A case study”, Water Science & Technology 57, 1555–1561.
Schyns, Z.O.G. and M.P. Shaver (2020), “Mechanical recycling of packaging plastics: a review”, Macromolecular Rapid Communications 42:2000415.
Scown, C.D. and J.D. Keasling (2022), “Sustainable manufacturing with synthetic biology”, Nature Biotechnology 40, 304-307.
Shelake, R.M., U.S. Kadam, R. Kumar, D. Pramanik, A.K. Singh and J.-Y. Kim (2022), “Engineering drought and salinity tolerance traits in crops through CRISPR-mediated genome editing: Targets, tools, challenges, and perspectives”, Plant Communications 3, 100417.
Siegmeier, T., B. Blumenstein and D. Möller (2015), “Farm biogas production in organic agriculture: System implications”, Agricultural Systems 139, 196-209.
Siles-Castellano, A.B., J.A. López-González, M.M. Jurado, M.J. Estrella-González, F. Suárez-Estrella and M.J. López (2021), “Compost quality and sanitation on industrial scale composting of municipal solid waste and sewage sludge”, Applied Sciences 11:7525.
Simkin, A.J., P.E. López-Calcagno and C.A. Raines (2019), “Feeding the world: improving photosynthetic efficiency for sustainable crop production”, Journal of Experimental Botany 70, 1119-1140.
Singh, P. and S.A. Ali (2021). Impact of CRISPR-Cas9-based genome engineering in farm animals. Vet Science 8:122.
Skocek, J., M. Zajac and M. Ben Haha (2020), “Carbon capture and utilization by mineralization of cement pastes derived from recycled concrete”, Scientific Reports 10:5614.
Skullestad, J.L., R.A. Bohne and J. Lohne (2016), “High-rise timber buildings as a climate change mitigation measure - a comparative LCA of structural system alternatives”, Energy Procedia 96, 112-123.
Snæbjörnsdóttir, S. Ó. and S.R. Gislason (2016), “CO2 Storage potential of basaltic rocks offshore Iceland” Energy Procedia 86, 371-380.
Solis, M. and S. Silveira (2020), “Technologies for chemical recycling of household plastics – A technical review and TRL assessment”, Waste Management 105, 128-138.
Song, Y., E. Ozdemir, S. Ramesh, A. Adishev, S. Subramanian, A. Harale, M. Albuali, B.A. Fadhel, A. Jamal, D. Moon, S.H. Choi and C.T. Yavuz (2020), “Dry reforming of methane by stable Ni–Mo nanocatalysts on single-crystalline MgO”, Science 367 (6479):777.
SOTACARBO (2020), “Methanol from CO2: Applications and Perspectives”, 14 May 14, 2020. www.sotacarbo.it/en/2020/05/metanolo-da-co2-applicazioni-e-prospettive/?fbclid=IwAR2P_f5qhTlLxqUy9pNEhgAU1FklyWvyAPJL99v_qzsfq4X6PGwnrNZI4i4/.
Springmann, M., M. Clark, D. Mason-D’Croz, K. Wiebe, B.L. Bodirsky, L. Lassaletta, W. de Vries, S.J. Vermeulen, M. Herrero, K.M. Carlson, M. Jonell, M. Troell, F. DeClerck, L.J. Gordon, R. Zurayk, P. Scarborough, M. Rayner, B. Loken, J. Fanzo, H.C.J. Godfray, D. Tilman, J. Rockström and W. Willett (2018), “Options for keeping the food system within environmental limits”, Nature 562, 519-525.
Tabashnik, B.E. and Y. Carrière (2017), “Surge in insect resistance to transgenic crops and prospects for sustainability”, Nature Biotechnology 35, 926-935.
Tickner, J., K. Geiser and S. Baima (2021), “Transitioning the chemical industry: the case for addressing the climate, toxics, and plastics crises”, Environment: Science and Policy for Sustainable Development 63, 4-15.
Tripathi, A., A. Jain, A.K. Singh, P. Choudhary, K.K. Mishra, P.C. Vashist (2022), Chapter 9 – “The Internet of Things in agriculture for sustainable rural development, In Intelligent Data-Centric Systems, AI, Edge and IoT-based Smart Agriculture”, (eds.) A. Abraham, S. Dash, J.J.P.C. Rodrigues, B. Acharya and S.K. Pani, pp.157-170, Academic Press, ISBN 9780128236949.
UK Department of Energy and Climate Change (2016), “Review of support for anaerobic digestion and micro-combined heat and power under the feed-in tariffs scheme”, UK Department of Energy and Climate Change, London.
UNDP (2021), “Peoples’ climate vote results”, United Nations Development Programme and University of Oxford, UK, www.undp.org/publications/peoples-climate-vote.
Van Gerrewey, T., N. Boon and D. Geelen (2021), “Vertical farming: the only way is up?”, Agronomy, 12:2.
Wen, A., K.L. Havens, S.E. Bloch, N. Shah, D.A. Higgins, A.G. Davis-Richardson, J. Sharon, F. Rezaei, M. Mohiti-Asli, A. Johnson, G. Abud, J.-M. Ane, J. Maeda, V. Infante, S.S. Gottlieb, J.G. Lorigan, L. Williams, A. Horton, M. McKellar, D. Soriano, Z. Caron, H. Elzinga, A. Graham, R. Clark, S.-M. Mak, L. Stupin, A. Robinson, N. Hubbard, R. Broglie, A. Tamsir and Karsten Temme (2021), “Enabling biological nitrogen fixation for cereal crops in fertilized fields”, ACS Synthetic Biology 10, 3264-3277.
Werner, C., H.-P. Schmidt, D. Gerten, W. Lucht and C. Kammann (2018), “Biogeochemical potential of biomass pyrolysis systems for limiting global warming to 1.5°C”, Environmental Research Letters 13:044036.
World Energy Council (2021), “International hydrogen strategies”, www.weltenergierat.de/wp-content/uploads/2020/09/WEC_H2_Strategies_finalreport_200922.pdf.
Youngok, K., Y. Eunkyung and S. Hyunik (2022), “Russia’s policy transition to a hydrogen economy and the implications of South Korea–Russia cooperation”, Energies 15:127, https://doi.org/10.3390/en15010127.
Zanuttini, R. and Negro, F. (2021), “Wood-based composites: innovation towards a sustainable future”, Forests 12, 1717. https://doi.org/10.3390/f12121717.
Zheng, Y.-L., H.-C. Liu and Y.-W. Zhang (2020), “Engineering heterostructured nanocatalysts for CO2 transformation reactions: advances and perspectives”, ChemSusChem 13, 6090-6123.
Zhu, Q. (2019), “Developments on CO2-utilization technologies”, Clean Energy 3, 85–100.
Zomer, R.J., D.A. Bossio, R. Sommer and L.V. Verchot (2017), “Global sequestration potential of increased organic carbon in cropland soils”, Scientific Reports 7:15554.
Notes
← 1. www.synthace.com/ Last accessed 1 March 2023.
← 2. www.bcg.com/ja-jp/publications/2022/combating-climate-crisis-with-alternative-protein Last accessed 1 March 2023.
← 3. www.usda.gov/media/blog/2018/08/14/vertical-farming-future Last accessed 1 March, 2023.
← 4. www.nesta.org.uk/feature/precision-agriculture/smart-tractors/ Last accessed 1 March 2023.
← 5. www.toastale.com/ Last accessed 1 March 2023.
← 6. https://onlinelibrary.wiley.com/doi/epdf/10.1002/bbb.2103 Last accessed 1 March 2023.
← 9. https://ourworldindata.org/land-use#:~:text=international%20data%20sources.-,Definitions%20of%20agricultural%20land%20use,%25%20of%20the%20Land%20Area) Last accessed 1 March 2023.
← 10. www.biobasedpress.eu/2021/06/meat-alternatives-on-the-rise/ Last accessed 1 March 2023.
← 11. https://bio.news/agriculture/biotech-makes-sustainable-plant-based-meat-tastier/ Last accessed 1 March 2023.
← 12. https://rumin8.com/how/ Last accessed 1 March 2023.
← 13. www.france24.com/en/tv-shows/talking-europe/20230407-if-we-don-t-solve-the-nitrogen-crisis-our-economy-will-get-stuck-dutch-deputy-pm.
← 14. https://public.wmo.int/en/media/news/number-of-wildfires-forecast-rise-50-2100 Last accessed 1 March 2023.
← 15. www.fao.org/3/i4373e/i4373e.pdf Last accessed 1 March 2023.
← 16. www.earthmicrobiome.org Last accessed 1 March 2023.
← 17. https://food.ec.europa.eu/horizontal-topics/farm-fork-strategy_en Last accessed 1 March 2023.
← 18. https://ec.europa.eu/clima/eu-action/forests-and-agriculture/sustainable-carbon-cycles/carbon-farming_en Last accessed 1 March 2023.
← 19. https://biochar-international.org/ Last accessed 1 March 2023.
← 20. www-erneuerbare--energien-de.translate.goog/EE/Redaktion/DE/Dossier/eeg.html?_x_tr_sl=de&_x_tr_tl=en&_x_tr_hl=en&_x_tr_pto=sc Last accessed 1 March 2023.
← 21. www.fdfa.be/en/climate Last accessed 1 March 2023.
← 22. www.ferc.gov/media/ferc-order-no-2222-fact-sheet Last accessed 1 March 2023.
← 24. A gate fee is the charge levied upon a given quantity of waste received at a waste processing facility. It is generally.
← 25. https://ec.europa.eu/eurostat/web/products-eurostat-news/-/ddn-20210216-1 Last accessed 1 March 2023.
← 27. https://thedriven.io/2022/01/14/shipping-giant-maersk-ups-order-for-green-methanol-powered-vessels/ Last accessed 1 March 2023.
← 28. www.carboncapturejournal.com/ViewNews.aspx?NewsID=4725 Last accessed 1 March 2023.
← 29. Cracking is the common term for a process that breaks up hydrocarbon molecules. In most cases this is carried out thermally, requiring large inputs of energy in the form of heat. Steam cracking is a specific cracking process used to convert hydrocarbons such as naphtha, liquified petroleum gases and natural gas condensates into olefins. The olefins are the monomers of the global plastics industry, essential to everyday life.
← 30. www.basf.com/global/en/who-we-are/sustainability/whats-new/sustainability-news/2022/basf-sabic-and-linde-start-construction-of-the-worlds-first-demonstration-plant-for-large-scale-electrically-heated-steam-cracker-furnaces.html Last accessed 1 March 2023.
← 31. https://carbonengineering.com/ Last accessed 1 March 2023.
← 32. https://carbonengineering.com/news-updates/multi-million-tonne-south-texas/ Last accessed 1 March 2023.
← 33. https://daccoalition.org/what-the-inflation-reduction-act-means-for-direct-air-capture/ Last accessed 1 March 2023.
← 34. https://climeworks.com/roadmap/orca Last accessed 1 March 2023.
← 35. https://epthinktank.eu/2022/02/23/new-eu-forest-strategy-for-2030/ Last accessed 1 March 2023.
← 36. www.npd.no/en/facts/publications/co2-atlases/co2-storage-atlas-norwegian-north-sea/ Last accessed 1 March 2023.
← 37. https://status22.globalccsinstitute.com/wp-content/uploads/2022/12/Global-Status-of-CCS-2022_Download_1222.pdf.
← 38. www.regjeringen.no/en/topics/energy/landingssider/ny-side/sporsmal-og-svar-om-langskip-prosjektet/id2863902/.
← 39. www.cleanenergyregulator.gov.au/ERF Last accessed 1 March 2023.
← 40. www.ice.org.uk/engineering-resources/case-studies/sleipner-carbon-capture-and-storage-project/ Last accessed 1 March 2023.
← 41. www.usgs.gov/news/featured-story/making-minerals-how-growing-rocks-can-help-reduce-carbon-emissions#:~:text=Carbon%20mineralization%20is%20the%20process,escape%20back%20to%20the%20atmosphere. Last accessed 1 March 2023.
← 42. www.canada.ca/en/environment-climate-change/services/climate-change/pricing-pollution-how-it-will-work/output-based-pricing-system.html.
← 43. www.jku.at/en/linz-institute-of-technology/research/research-facilities/cleanroom/cleanroom-at-the-lit-open-innovation-center/ Last accessed 1 March 2023.
← 44. www.co2exide.eu/ Last accessed 1 March 2023.
← 45. www.jku.at/en/institute-of-physical-chemistry-and-linz-institute-for-organic-solar-cells/research/projects/enzymbiokat/ Last accessed 1 March 2023.
← 46. www.frontiersin.org/research-topics/15676/nanomaterials-for-carbon-dioxide-capture-and-utilization#overview Last accessed 1 March 2023.
← 47. https://cordis.europa.eu/article/id/418045-how-to-develop-nano-based-structures-for-drag-reduction-in-aviation-and-manufacturing Last accessed 1 March 2023.
← 48. www.energy.gov/eere/fuelcells/hydrogen-production-electrolysis Last accessed 1 March 2023.
← 49. www.rystadenergy.com/insights/hydrogen-report-january Last accessed 1 March 2023.
← 50. https://epthinktank.eu/2022/05/31/refueleu-aviation-initiative-summary-of-the-commission-proposal-and-the-parliaments-draft-committee-report/ Last accessed 1 March 2023.
← 51. https://ptx-hub.org/delegated-acts-on-art-27-and-28-explained/ Last accessed 1 March 2023.